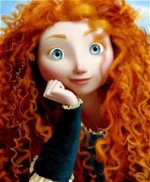
Use of Water Properties in Food Technology: A Global View
Water is a major component of drinking water, beverages, and most foodstuffs. In this study, an effort has been made to employ selected properties of water for: (1) evaluation of interactions of water with other food components; (2) discussion on the effects of water properties on food and beverage products; (3) applications of water properties in food technology; and (4) comparison of water properties with corresponding properties of similar substances. This study provides the following major conclusions: (i) unusual properties of water are mostly due to its high permanent dipole moment, partial ionic character of O–H covalent bonds, and extensive hydrogen bonds; (ii) different properties of many foodstuffs are strongly related to various properties of water; (iii) the properties of food products change depending on water availability and temperature; (iv) preparation of drinking water is a prerequisite for production of any safe drinks and foodstuffs; (v) water contributes important roles in quality, flavor, and shelf-life of foods; and (vi) water is used in food industries as a fluid for heat transfer; as a medium for temperature moderation in food processing; as a solvent for sugars, salts, water-soluble vitamins, and acids; as a dispersing agent for hydrophilic food components; as a dispersed phase for emulsified products; or as a reactant for several reactions in food processing.
INTRODUCTION
Water is the only substance on the Earth that occurs in all three physical states simultaneously (solid/ice, liquid/water, and gas/water vapor). It is an essential material for life: living systems consist of water and its amount is about 70–80% (w/w). Water possesses many chemical and physical properties that make it useful to cells and organisms. It is a medium for the majority of biochemical reactions.[1,2]
Water is a nutrient in food groups: grains, meats, dairy products, fruits, and vegetables. Major nutrients such as carbohydrates, proteins, water-soluble vitamins, and minerals are hydrophilic. Most of carbohydrates and proteins in foods are plasticized by water. A part of lipid components are hydrophilic, whereas another part of lipid and protein components are hydrophobic and experience from hydrophilic effect in an aqueous environment.[1,2] Water interacts with other food components by means of polar, hydrogen-bonding, and hydrophobic interactions. These interactions change the properties of water.
Water plays many critical roles within the field of agriculture, food, and feed science, technology, and engineering. Water is the main components of drinking water, beverages and most of foodstuffs. Water content of fresh fruits, vegetables, meats, and sea foods exceeds 50%. Water is used as a good medium to cook foods. In addition, steam is the basic working medium of heat (as a feed for boiler) and power engineering in food processing. In food chains, water is not just a medium for reactions, but is also an active ingredient used to control reactions, food texture, and physical and biological behavior. Food may pick up moisture from the environment or lose moisture to the environment during storage. The percent of loss of fresh foods can be significantly reduced by controlling their water activity during storage. These changes may influence the texture and organoleptic properties of foodstuffs. Many operations in food processing (concentration, drying, dehydration, thawing, salting, and freezing) use physico-chemical properties of water and its state transition properties.[2–6]
Significant amounts of information on the properties of water are available in the literature. The information and its importance to food technology are dispersed and fragmented. The objectives of this study are to describe various properties of water, to discuss the effects of water properties on individual food components and combined foods, to describe its importance to food technology, and to compare properties of water with corresponding properties of similar substances. Several properties of water (boiling and melting points, heat of fusion, heat of vaporization and sublimation, heat capacity, thermal conductivity, surface tension, and viscosity) have been compared with similar substances.The information given in this study can be used for processing, storage, and packaging of beverages and foodstuffs.
DESCRIPTION OF CHEMICAL STRUCTURE, MORPHOLOGY, AND PHASE DIAGRAM OF WATER
Chemical Structure
The structural, physical, and chemical parameters for liquid and solid states of water are given in Tables 1 and 2.[1–7] A single H2O molecule is a polar molecule with a dipole moment of 1.85 Debye (D).[7] An individual H2O molecule has approximately tetrahedral geometry, with an oxygen atom at the center of a tetrahedron, two hydrogen atoms at two corners, and two lone pair electrons at two other corners. The molecule oriented along H–O–H linkage with an average angle of 104.5° is slightly smaller than the tetrahedral angle (109.5°). The positive charge density of the molecule is localized on the hydrogen and negative charge on the oxygen atoms.[8–10] The water molecule possesses partial ionic character due to the highly electro-negative oxygen.[10–12] Water molecules have a two-fold rotation axis and two mirror planes of symmetry. These give the water molecules a symmetry point group C2v.[13]
The distribution of electrons in a single water molecule is different from those of dimers, clusters, and bulk water.[13] The dipole moments of liquid and solid states of water appear to be higher than that of a single H2O molecule.[13] The dipole moment in the liquid water was reported to be between 2 and 4 D, depending on the nature of water-water interactions.[10] The negatively charged region of one molecule is attracted to the positively charged region of another molecule by electrostatic forces.[10,11] At room temperature (20°C), its total free energy of cohesion (ΔC cohesion = -145.6 mJ.m2) consisting of polar (ΔG polar = -102 mJ.m2), and van der Waals (ΔG van der Waals = -43.6 mJ.m2). The polar and van der Waals part of the free energies of cohesion represent 70 and 30% of the total energy, respectively.[12]
The structure of water in the liquid and solid states is based on long-ranged interactions (hydrogen-bonding attractions), and short-ranged repulsions.[14] Water molecules associate via hydrogen bonds and form a three-dimensional structure.[11,14,15] There is a vacant space between water molecules. Water can act twice as a proton donor and twice as an electron donor (two alone electron pairs of the O atom). The H-bond interactions in the liquid water constitute a 3–D–H– bond network with localized and structured clustering.[10,14,15] The number of neighboring water molecules for a water molecule in the liquid water is between 4 and 5.[2,4,14,15] At room temperature, the average number of neighboring water molecules for a molecule in the liquid water is 4.5. An increase in temperature causes a decrease in the cluster's size and an increase in the electron-acceptor ability.[12] The cluster's size increases close to 0°C, whereas the size decreases near boiling point.[6]
The dissociation energy for hydrogen bonds in pure water equals to 20.0 kJ.mol−1,[4,6,7] which is greater than that of the fluctuating dipole energy in the noble gases (2.1–6.3 kJ.mol−1).[16] In water-water H-bonding interactions due to mutual polarization effects, the strength of H-bonds enhances by 20%.[10] Hydrogen bond energies formed between water and other substances have been reported to be a wide range (12.6–33.5 kJ. mol−1).[15,17] Electrostatic force makes a major contribution to the energy of the hydrogen bonds. The hydrogen bonds’ lengths are somewhat longer than that of covalent bonds; their OH … distance ranges from 1.5 to 2.6 Å.[6] The dissociation energy for O–H covalent bond in pure water is 462.3 kJ.mol−1. Hydrogen bonds have greater variation of lengths in comparison with covalent bonds.
Formation of hydrogen bonds enhances the polarity of O–H bonds, and consequently the intensity of corresponding infrared bands, IR, 3300 cm−1 increases.[18,19] The spectrum of water depends on temperature and density of water.[13] A water molecule has three normal modes of vibration (ν1,ν2,ν3). Two modes of vibration, symmetric stretching (ν1 = 3656 cm−1) and asymmetric stretching (ν3 = 3756 cm−1) are nearly along the direction of the O–H bonds. The symmetric stretching vibration involves a small amount of H–O–H bending. The mode ν2 (ν2 = 1594 cm−1) is bending (or deformation) and it is almost perpendicular to the direction of the O–H bonds and this mode of vibration involves a small amount of O–H stretching.[13]
Formation of hydrogen bonds strongly affects the chemical shift values for proton nuclei (δ H ) in nuclear magnetic resonance (NMR) spectra. The hydrogen bond formation leads to a decrease in shielding of the protons and the value of δ H has been shifted toward a weak magnetic field. The value of δ H for a proton of a single water molecule is practically independent of pressure and temperature, but for hydrogen bonded complexes, the opposite effect was observed. For example, distortion of a portion of the hydrogen bonds with an increase in temperature results in shifting of the proton resonance toward stronger magnetic field.[20,21]
Water molecules interact strongly with one another through hydrogen bonds. In the structure of ice, these interactions are apparent; networks of hydrogen bonds hold the structure together.[11–13] In the liquid state, similar interactions between water molecules via hydrogen bonds are formed. However, in the liquid state some of hydrogen bonds are broken. The highly cohesive nature of water dramatically affects the interaction between molecules in aqueous solution.[11,13]
Different substances may interact with water in six ways (via hydrogen bonding, ionic bonding, hydrophobic association, London dispersion or van der Waals forces, the effect of trapping water in polymer capillaries, and solute effects due to entropy considerations).[2,8,10,13,22–24] The polarity and hydrogen-bonding capability of water make it a highly interacting molecule. The structure of water in a solution is disordered slightly in comparison with the structure of pure water. The influence of ions on the structure of water can be considered as the sum of two different actions: (1) formation of solvation shells, which is accompanied by compression and a reduction of entropy; and (2) destruction of molecular structure of water by the ions, especially large ones and at high concentrations, which leads to an increase in its entropy.[25]
States and Morphologies of Water
Bulk and interfacial water can be described in terms of a two-state mixture model including high density water (HDW), with collapsed or condensed structure (ρ > 1 g.cm−3) under standard conditions, and low-density water (LDW) with expanded structure (ρ < 1 g.cm−3) being in the dynamic equilibrium.[20] The hydrogen bond network in HDW is distorted in comparison with that of ice [δ H = 7 ppm for ideal LDW]. The value of δ H decreases to δ H = 4.8 ppm (HDW) under standard conditions.[21,26]
When water converts to ice, it gains a crystalline-like or amorphous (glassy) structure. The structure of ice crystals is a series of parallel planes and has a hexagonal symmetry. In this form, nearly all of the water molecules are joined by the maximum number of hydrogen bonds. Hydrogen bond is prominent in the crystal structures of various solid phases of water. In ice (common in terms of pressure and temperature), the tetrahedral structure of water molecules leads to four hydrogen bonds: two as donors (from hydrogen) and two as acceptors (from oxygen). Li and Ross[27] have shown that two different types of hydrogen bonds with different strengths, strong and weak in the ratio of about 2:1, are randomly distributed throughout the network of ice using inelastic neutron-scattering spectra.
There are at least 11 different forms of crystalline ice that are known so far. The ordinary hexagonal ice, which is usually designated as Ih, is the only one that is found naturally. It is a stable polymorph of ice at atmospheric conditions, and is an ideal representation of hydrogen-bonded network.[11,27,28] When liquid water freezes slowly at 0°C under atmospheric or lower pressure, the molecules have a chance of arranging into the above-mentioned structure.[27] In ice Ih, each water molecule interacts tetrahedrally with four other molecules via hydrogen bonds with O–O distances of 2.76 Å to the nearest oxygen neighbor. The O–O–O angles are 109°, typical of a tetrahedrally coordinated lattice structure.[29]
When liquid water is frozen rapidly, the molecules have little chance of arranging into crystalline ice and results in an amorphous structure. A fast freezing rate prevents the migration of water into the extracellular spacing. Generally, a high freezing rate is desired in order to obtain numerous fine ice crystals. Nevertheless, this is not always the case.[30] For example, consider frozen dough, for which a slower freezing gives a better preservation of yeast activity. A slow rate results in cell dehydration and large ice crystals that might damage the texture of a food. The difference in the effects of fast and slow freezing of foods could be analyzed with respect to the characteristics and the structure of formed ice.
From a physical point of view, foods (land animals, fishes, fruits, vegetables) can be roughly considered as dilute aqueous solutions. Ice formation in foods generally occurs between −1 and −3°C depending on the nature and molar concentration of dissolved substances presented.[31] Ice formation occurs during freezing only after a certain degree of super-cooling. As the products are progressively cooled below their initial freezing point, more water will be turned into ice and the solution will become more concentrated.[31]
Strong hydrogen bonds make ice hard, but brittle. The structure of ice provides a striking example of many hydrogen bonds. X-ray and neutron studies have established that water molecules in ice are arranged in an unusually open structure.[10] Formation of hydrogen bond in ice causes to increase in O–H covalent bond length (from 0.96 Å to 1.0 Å).[10]
Phase Diagram for Water
One can use phase diagram to predict changes in melting and boiling points of a substance as a result of changes in the external pressure. In general, phase boundary line for conversion of a solid into liquid has a positive slope. If the volume change is negative, the phase boundary line has an opposite slope, as happens for the melting of water (phase diagram was not shown).[15,16,32] Consuming energy, releasing energy, and changing in pressure can cause phase changes. The higher the external pressure, the lower the melting point. The changes in molar entropies (ΔS), molar volumes (ΔV), and enthalpies ((ΔH), for conversion of ice into liquid water are given in Table 3.[1–7] The solid/liquid transition is accompanied by discontinuities in many physical properties, e.g., density, internal energy, and heat capacity. At high pressures differences in the properties of the solid and the liquid remain unchanged, whereas those of the liquid and the gas decrease and merge at the critical point.[11] The transition of water from liquid into solid plays an important role in storage and packaging of foods.
The transition of water from liquid into solid plays an important role in storage and packaging of foods.
Density-Temperature-Volume Relationship
The relation between the density of a liquid and temperature is usually expressed by a linear equation as follows:[3,40](1)where ρ t , ρ 0, and k are densities of the liquid at T and T 0 and coefficient of thermal expansion of the liquid, respectively. The density of the liquid monotonically decreases as a function of temperature at constant pressure. An exception of the general law is water. The density of ice and water as a function of temperature was given in Fig. 1a. Between 3.98°C (˜4°C) and the freezing point, 0°C, the liquid water gradually expands and becoming less dense structure (Fig. 1b), similar to the ordinary ice.[40,41] The variation of volume as a function of temperature at atmospheric pressure, , consists of two components as follows:(2)The first term of the right-hand side of Eq. (2) corresponds to the thermal expansion of the liquid. This term is always positive. It varies only slightly with temperature and may be considered constant for a small temperature interval. The second term of the right-hand side of Eq. (2) corresponds to the change in volume caused by the rupture of bonds between molecules with an increase in temperature.[40] The melting process of ice is accompanied by a decrease in volume, and for this reason the second term is negative. The latter effect become
Use of Water Properties in Food Technology: A Global View
Abstract
Water is a major component of drinking water, beverages, and most foodstuffs. In this study, an effort has been made to employ selected properties of water for: (1) evaluation of interactions of water with other food components; (2) discussion on the effects of water properties on food and beverage products; (3) applications of water properties in food technology; and (4) comparison of water properties with corresponding properties of similar substances. This study provides the following major conclusions: (i) unusual properties of water are mostly due to its high permanent dipole moment, partial ionic character of O–H covalent bonds, and extensive hydrogen bonds; (ii) different properties of many foodstuffs are strongly related to various properties of water; (iii) the properties of food products change depending on water availability and temperature; (iv) preparation of drinking water is a prerequisite for production of any safe drinks and foodstuffs; (v) water contributes important roles in quality, flavor, and shelf-life of foods; and (vi) water is used in food industries as a fluid for heat transfer; as a medium for temperature moderation in food processing; as a solvent for sugars, salts, water-soluble vitamins, and acids; as a dispersing agent for hydrophilic food components; as a dispersed phase for emulsified products; or as a reactant for several reactions in food processing.
Keywords: Water, Properties, Food applications
INTRODUCTION
Water is the only substance on the Earth that occurs in all three physical states simultaneously (solid/ice, liquid/water, and gas/water vapor). It is an essential material for life: living systems consist of water and its amount is about 70–80% (w/w). Water possesses many chemical and physical properties that make it useful to cells and organisms. It is a medium for the majority of biochemical reactions.[1,2]
Water is a nutrient in food groups: grains, meats, dairy products, fruits, and vegetables. Major nutrients such as carbohydrates, proteins, water-soluble vitamins, and minerals are hydrophilic. Most of carbohydrates and proteins in foods are plasticized by water. A part of lipid components are hydrophilic, whereas another part of lipid and protein components are hydrophobic and experience from hydrophilic effect in an aqueous environment.[1,2] Water interacts with other food components by means of polar, hydrogen-bonding, and hydrophobic interactions. These interactions change the properties of water.
Water plays many critical roles within the field of agriculture, food, and feed science, technology, and engineering. Water is the main components of drinking water, beverages and most of foodstuffs. Water content of fresh fruits, vegetables, meats, and sea foods exceeds 50%. Water is used as a good medium to cook foods. In addition, steam is the basic working medium of heat (as a feed for boiler) and power engineering in food processing. In food chains, water is not just a medium for reactions, but is also an active ingredient used to control reactions, food texture, and physical and biological behavior. Food may pick up moisture from the environment or lose moisture to the environment during storage. The percent of loss of fresh foods can be significantly reduced by controlling their water activity during storage. These changes may influence the texture and organoleptic properties of foodstuffs. Many operations in food processing (concentration, drying, dehydration, thawing, salting, and freezing) use physico-chemical properties of water and its state transition properties.[2–6]
Significant amounts of information on the properties of water are available in the literature. The information and its importance to food technology are dispersed and fragmented. The objectives of this study are to describe various properties of water, to discuss the effects of water properties on individual food components and combined foods, to describe its importance to food technology, and to compare properties of water with corresponding properties of similar substances. Several properties of water (boiling and melting points, heat of fusion, heat of vaporization and sublimation, heat capacity, thermal conductivity, surface tension, and viscosity) have been compared with similar substances.The information given in this study can be used for processing, storage, and packaging of beverages and foodstuffs.
DESCRIPTION OF CHEMICAL STRUCTURE, MORPHOLOGY, AND PHASE DIAGRAM OF WATER
Chemical Structure
The structural, physical, and chemical parameters for liquid and solid states of water are given in Tables 1 and 2.[1–7] A single H2O molecule is a polar molecule with a dipole moment of 1.85 Debye (D).[7] An individual H2O molecule has approximately tetrahedral geometry, with an oxygen atom at the center of a tetrahedron, two hydrogen atoms at two corners, and two lone pair electrons at two other corners. The molecule oriented along H–O–H linkage with an average angle of 104.5° is slightly smaller than the tetrahedral angle (109.5°). The positive charge density of the molecule is localized on the hydrogen and negative charge on the oxygen atoms.[8–10] The water molecule possesses partial ionic character due to the highly electro-negative oxygen.[10–12] Water molecules have a two-fold rotation axis and two mirror planes of symmetry. These give the water molecules a symmetry point group C2v.[13]
Table 1 Structural and physicochemical parameters for the liquid water
Table 2 Density and thermal properties of solid water (ice) at 0°C and −20°C
The distribution of electrons in a single water molecule is different from those of dimers, clusters, and bulk water.[13] The dipole moments of liquid and solid states of water appear to be higher than that of a single H2O molecule.[13] The dipole moment in the liquid water was reported to be between 2 and 4 D, depending on the nature of water-water interactions.[10] The negatively charged region of one molecule is attracted to the positively charged region of another molecule by electrostatic forces.[10,11] At room temperature (20°C), its total free energy of cohesion (ΔC cohesion = -145.6 mJ.m2) consisting of polar (ΔG polar = -102 mJ.m2), and van der Waals (ΔG van der Waals = -43.6 mJ.m2). The polar and van der Waals part of the free energies of cohesion represent 70 and 30% of the total energy, respectively.[12]
The structure of water in the liquid and solid states is based on long-ranged interactions (hydrogen-bonding attractions), and short-ranged repulsions.[14] Water molecules associate via hydrogen bonds and form a three-dimensional structure.[11,14,15] There is a vacant space between water molecules. Water can act twice as a proton donor and twice as an electron donor (two alone electron pairs of the O atom). The H-bond interactions in the liquid water constitute a 3–D–H– bond network with localized and structured clustering.[10,14,15] The number of neighboring water molecules for a water molecule in the liquid water is between 4 and 5.[2,4,14,15] At room temperature, the average number of neighboring water molecules for a molecule in the liquid water is 4.5. An increase in temperature causes a decrease in the cluster's size and an increase in the electron-acceptor ability.[12] The cluster's size increases close to 0°C, whereas the size decreases near boiling point.[6]
The dissociation energy for hydrogen bonds in pure water equals to 20.0 kJ.mol−1,[4,6,7] which is greater than that of the fluctuating dipole energy in the noble gases (2.1–6.3 kJ.mol−1).[16] In water-water H-bonding interactions due to mutual polarization effects, the strength of H-bonds enhances by 20%.[10] Hydrogen bond energies formed between water and other substances have been reported to be a wide range (12.6–33.5 kJ. mol−1).[15,17] Electrostatic force makes a major contribution to the energy of the hydrogen bonds. The hydrogen bonds’ lengths are somewhat longer than that of covalent bonds; their OH … distance ranges from 1.5 to 2.6 Å.[6] The dissociation energy for O–H covalent bond in pure water is 462.3 kJ.mol−1. Hydrogen bonds have greater variation of lengths in comparison with covalent bonds.
Formation of hydrogen bonds enhances the polarity of O–H bonds, and consequently the intensity of corresponding infrared bands, IR, 3300 cm−1 increases.[18,19] The spectrum of water depends on temperature and density of water.[13] A water molecule has three normal modes of vibration (ν1,ν2,ν3). Two modes of vibration, symmetric stretching (ν1 = 3656 cm−1) and asymmetric stretching (ν3 = 3756 cm−1) are nearly along the direction of the O–H bonds. The symmetric stretching vibration involves a small amount of H–O–H bending. The mode ν2 (ν2 = 1594 cm−1) is bending (or deformation) and it is almost perpendicular to the direction of the O–H bonds and this mode of vibration involves a small amount of O–H stretching.[13]
Formation of hydrogen bonds strongly affects the chemical shift values for proton nuclei (δ H ) in nuclear magnetic resonance (NMR) spectra. The hydrogen bond formation leads to a decrease in shielding of the protons and the value of δ H has been shifted toward a weak magnetic field. The value of δ H for a proton of a single water molecule is practically independent of pressure and temperature, but for hydrogen bonded complexes, the opposite effect was observed. For example, distortion of a portion of the hydrogen bonds with an increase in temperature results in shifting of the proton resonance toward stronger magnetic field.[20,21]
Water molecules interact strongly with one another through hydrogen bonds. In the structure of ice, these interactions are apparent; networks of hydrogen bonds hold the structure together.[11–13] In the liquid state, similar interactions between water molecules via hydrogen bonds are formed. However, in the liquid state some of hydrogen bonds are broken. The highly cohesive nature of water dramatically affects the interaction between molecules in aqueous solution.[11,13]
Different substances may interact with water in six ways (via hydrogen bonding, ionic bonding, hydrophobic association, London dispersion or van der Waals forces, the effect of trapping water in polymer capillaries, and solute effects due to entropy considerations).[2,8,10,13,22–24] The polarity and hydrogen-bonding capability of water make it a highly interacting molecule. The structure of water in a solution is disordered slightly in comparison with the structure of pure water. The influence of ions on the structure of water can be considered as the sum of two different actions: (1) formation of solvation shells, which is accompanied by compression and a reduction of entropy; and (2) destruction of molecular structure of water by the ions, especially large ones and at high concentrations, which leads to an increase in its entropy.[25]
States and Morphologies of Water
Bulk and interfacial water can be described in terms of a two-state mixture model including high density water (HDW), with collapsed or condensed structure (ρ > 1 g.cm−3) under standard conditions, and low-density water (LDW) with expanded structure (ρ < 1 g.cm−3) being in the dynamic equilibrium.[20] The hydrogen bond network in HDW is distorted in comparison with that of ice [δ H = 7 ppm for ideal LDW]. The value of δ H decreases to δ H = 4.8 ppm (HDW) under standard conditions.[21,26]
When water converts to ice, it gains a crystalline-like or amorphous (glassy) structure. The structure of ice crystals is a series of parallel planes and has a hexagonal symmetry. In this form, nearly all of the water molecules are joined by the maximum number of hydrogen bonds. Hydrogen bond is prominent in the crystal structures of various solid phases of water. In ice (common in terms of pressure and temperature), the tetrahedral structure of water molecules leads to four hydrogen bonds: two as donors (from hydrogen) and two as acceptors (from oxygen). Li and Ross[27] have shown that two different types of hydrogen bonds with different strengths, strong and weak in the ratio of about 2:1, are randomly distributed throughout the network of ice using inelastic neutron-scattering spectra.
There are at least 11 different forms of crystalline ice that are known so far. The ordinary hexagonal ice, which is usually designated as Ih, is the only one that is found naturally. It is a stable polymorph of ice at atmospheric conditions, and is an ideal representation of hydrogen-bonded network.[11,27,28] When liquid water freezes slowly at 0°C under atmospheric or lower pressure, the molecules have a chance of arranging into the above-mentioned structure.[27] In ice Ih, each water molecule interacts tetrahedrally with four other molecules via hydrogen bonds with O–O distances of 2.76 Å to the nearest oxygen neighbor. The O–O–O angles are 109°, typical of a tetrahedrally coordinated lattice structure.[29]
When liquid water is frozen rapidly, the molecules have little chance of arranging into crystalline ice and results in an amorphous structure. A fast freezing rate prevents the migration of water into the extracellular spacing. Generally, a high freezing rate is desired in order to obtain numerous fine ice crystals. Nevertheless, this is not always the case.[30] For example, consider frozen dough, for which a slower freezing gives a better preservation of yeast activity. A slow rate results in cell dehydration and large ice crystals that might damage the texture of a food. The difference in the effects of fast and slow freezing of foods could be analyzed with respect to the characteristics and the structure of formed ice.
From a physical point of view, foods (land animals, fishes, fruits, vegetables) can be roughly considered as dilute aqueous solutions. Ice formation in foods generally occurs between −1 and −3°C depending on the nature and molar concentration of dissolved substances presented.[31] Ice formation occurs during freezing only after a certain degree of super-cooling. As the products are progressively cooled below their initial freezing point, more water will be turned into ice and the solution will become more concentrated.[31]
Strong hydrogen bonds make ice hard, but brittle. The structure of ice provides a striking example of many hydrogen bonds. X-ray and neutron studies have established that water molecules in ice are arranged in an unusually open structure.[10] Formation of hydrogen bond in ice causes to increase in O–H covalent bond length (from 0.96 Å to 1.0 Å).[10]
Phase Diagram for Water
One can use phase diagram to predict changes in melting and boiling points of a substance as a result of changes in the external pressure. In general, phase boundary line for conversion of a solid into liquid has a positive slope. If the volume change is negative, the phase boundary line has an opposite slope, as happens for the melting of water (phase diagram was not shown).[15,16,32] Consuming energy, releasing energy, and changing in pressure can cause phase changes. The higher the external pressure, the lower the melting point. The changes in molar entropies (ΔS), molar volumes (ΔV), and enthalpies ((ΔH), for conversion of ice into liquid water are given in Table 3.[1–7] The solid/liquid transition is accompanied by discontinuities in many physical properties, e.g., density, internal energy, and heat capacity. At high pressures differences in the properties of the solid and the liquid remain unchanged, whereas those of the liquid and the gas decrease and merge at the critical point.[11] The transition of water from liquid into solid plays an important role in storage and packaging of foods.
Table 3 Thermodynamic constants for phase changes of H2O
DESCRIPTION OF SEVERAL PROPERTIES OF WATER
General Considerations
The branch of water science employed in food science covers a vast range, including agriculture, chemistry, physics, biology, biochemistry, cookery, microbiology, nutrition, and power generation. Drinking water is used for processing, washing, cooling, and heating. Food processing operations includes preparation, formulation, and preservation (preparation: to convert raw food materials into edible ingredients; assembly or formulation: to combine food ingredients and produce foods; and preservation: to prevent spoilage of food products). Water influences food structures, appearances, flavor, and their susceptibility to spoilage.[2,5,15] Water maintains biological activities of proteins, nucleotides, and carbohydrates, and participates in hydrolysis and other chemical reactions.[2,5,15] Water is a major component of fruits, vegetables, meats, sea foods, and beverages (mineral water, carbonated and noncarbonated water, fruit and vegetable juices, soft drinks, spirits, coffee, tea, milk, and alcoholic drinks). A diluted fruit juice contains added water. Knowledge on the properties of water near or at transition temperatures (freezing and boiling) and ambient temperature are important to food technology, thus, the important parameters are given in Table 4.
Table 4 Physicochemical values for the liquid water at different temperatures
Franks[11] has called water a matrix of life. The author of this study believes that water is the most valuable material occurring in the nature. It is the most important component of microorganisms, organisms, foods, and feeds. It is an environmental friendly material and it is a safe solvent and a safe medium for several chemical and biochemical reactions. The production of safe drinking water is a prerequisite for production of any drinks and foods.
When water is associated with foods, it can be placed into three categories: free, absorbed (affected water), and bound water.[2–5,15] Water in narrow pores can be bound and it can be nearly free in macro-pores. Free water is frozen at 0°C but water bound in pores is frozen at lower temperatures. The deposited water on the surface of macromolecules (starches, pectin, cellulose, and proteins) is called absorbed water and results in formation of colloidal systems. This absorption is due to hydrogen bonding and van der Waals’ forces. Bound water (structural water or non-freezing water) is in combination with constituents of foodstuffs.[2–6,15] The properties of bound water depend on the chemical and spatial characteristics of the materials surrounded by water.[26] Bound water at the hydrophilic/hydrophobic interfaces can be assigned as weakly or strongly bound to the surfaces of food components. Liu and Yao[33] proposed that the nano-cavity in polymers is an important reason for the formation of unfrozen water. Unfrozen bound water contributes favorably to the conformational stability of protein.[34] The activities of enzymes depend upon the population of unfrozen bound water.[35] NMR studies of protein solutions have demonstrated that a certain amount of water does not freeze at 273 K.[26] The 1H NMR method, with layer-by-layer freezing of bulk water, is an appropriate method to analyze the behavior of the unusual interfacial water.[26] Bulk water (or free water in foodstuffs) and bound water have very different behaviors as has been illustrated by 1H NMR spectroscopy.[36]
Pectic substances and hydrocolloids (guar, locust bean, gum Arabic, tragacanth, agar, carrageenan, aliginate, dextran, and xanthan) are able to absorb or entrap large quantities of water. Water-holding capacity has been used to describe the ability of matrix molecules to entrap a large amount of water in such a manner that exudation is prevented.[2] Although the entrapped water does not flow freely from food components, it behaves like pure water during processing. Water-holding capacity of hydrocolloids and gels has a profound effect on rheological properties of foods. It is affected by pH, salts, and sugars. Low quantity of hydrocolloids or pectic substances in water can form gel. These polysaccharides can be used to make gels or to modify food texture. The properties of foodstuffs depend strongly on the nature and the quality of hydrocolloid-water interactions.
Most food components are characterized by alternate structures with hydrophilic and hydrophobic properties. A protein contains certain amino acids with both hydrophilic and hydrophobic side groups, and water stabilizes the protein structure. Polar and nonpolar fraction of food components are neighbors in macromolecules (proteins and carbohydrates). Polar compounds and ions (water and metal cations) are neighbors of either nonpolar components (lipids) or nonpolar fraction of food components (nonpolar side groups of proteins and carbohydrates or CH chains in phospholipids). The polar attraction between water in the liquid state causes the strong interactions between all hydrophobic molecules (hydrophobic effect) immersed in water. In contrast, the polar attraction between water molecules and hydrophilic substances causes the hydration pressure. Therefore, one can assume that water molecules are observed at mosaic hydrophobic/hydrophilic surfaces of different food components.[22–24]
In the processes of cooking, sterilization, concentration, dehydration, drying, or freezing of food chains, several water properties relating to heat transfer (heat capacity, latent heat of fusion and vaporization, thermal conductivity, viscosity, etc.) are involved.[3] When different chemical and biochemical materials are introduced into water, different properties of water are also involved.
Food quality as well as flavor of foods is a function of concentrations of food components (with emphasize on water). The interactions of food components and packaging materials include uptake of the major food constituents (water, fats, or oils).[37–39] The food packaging industry needs to understand the transport of water through packaging materials (synthetic and natural polymers). The interactions of water and packaging materials affect food quality as well as its organoleptic properties (flavor characteristics). The primary goal of food packaging is to protect the quality of foodstuffs. To meet this goal, the transport of water must be properly taken into account in material selection and design of food packaging containers. In storage and packaging of foodstuffs, especially fresh foods (meats, sea foods, fruits, vegetables) using modified atmosphere or controlled atmosphere, several properties of water should be also taken into account.
Density-Temperature-Volume Relationship
The relation between the density of a liquid and temperature is usually expressed by a linear equation as follows:[3,40](1)where ρ t , ρ 0, and k are densities of the liquid at T and T 0 and coefficient of thermal expansion of the liquid, respectively. The density of the liquid monotonically decreases as a function of temperature at constant pressure. An exception of the general law is water. The density of ice and water as a function of temperature was given in Fig. 1a. Between 3.98°C (˜4°C) and the freezing point, 0°C, the liquid water gradually expands and becoming less dense structure (Fig. 1b), similar to the ordinary ice.[40,41] The variation of volume as a function of temperature at atmospheric pressure, , consists of two components as follows:(2)The first term of the right-hand side of Eq. (2) corresponds to the thermal expansion of the liquid. This term is always positive. It varies only slightly with temperature and may be considered constant for a small temperature interval. The second term of the right-hand side of Eq. (2) corresponds to the change in volume caused by the rupture of bonds between molecules with an increase in temperature.[40] The melting process of ice is accompanied by a decrease in volume, and for this reason the second term is negative. The latter effect becomes weaker with an increase in temperature. At a temperature below 4°C, the second term predominates over the first one. Thus, the total amount of in this temperature region is negative, and decreases its value as the temperature approaches 4°C. At 4°C, both terms on the right-hand side are equal in absolute value and opposite in sign (the sign of thermal expansion coefficient changes from negative to positive), thus at 4°C:(3)
Figure 1 (a) Density of ice and water as a function of temperature; (b) density of the liquid water as a function of temperature (between 0 and 10°C). (This material is reproduced with permission of John Wiley & Sons. Morgan, J., Stumm, W., and Hem, J. Kirk-Othmer Encyclopedia of Chemical Technology, 5th Ed., John Wiley & Sons, 1969.)
Above 4°C, the first term predominates, and becomes positive and continues to increase with an increase in temperature. The temperature corresponding to the maximum density of water depends on pressure. For instance, at 10 atmosphere, it is 3.4°C, and at 145 atmosphere, it drops to 0.6°C. The plot of water density versus temperature deviates from its normal behavior, which is above 0°C and results in maximum density at 4°C. Makkonen[42] has explained why water reaches to a maximum density at above its freezing point as follows: a surface energy of 6.95 mJ.m−2 is related to the ice/water interface at equilibrium causing a surface pressure of 110 MPa and resulting in melting temperature change ΔT = −8°C. Thus, the equilibrium temperature at the ice/water interface is 8°C lower than the theoretical melting temperature of bulk ice. In conclusion, the theoretical melting temperature of bulk ice as well as the freezing temperature of bulk water is approximately 8°C.[42]
In contrast to the melting of most other substances, the melting of ice is accompanied by a decrease in volume. The density of liquid water and solid water (ice) at 0°C equals to 0.99989 g.cm−3 and 0.9165 g.cm−3,[7] respectively. The expansion of volume upon phase conversion from liquid water to ice equals to:(4)which is quite considerable. Thus, one should take into account the expansion volume for storage and packaging of foodstuffs (drinking water and beverages, meats, and sea foods) under freezing conditions. A container made of glass containing drinking water or beverage may burst under frozen conditions. Expansion of water during freezing may damage plastic packaging materials, some food industrial apparatus, and pipes. Water pipes may burst when water freezes inside them. The molar volume at 4°C is a minimum value (the density is maximum). The change in molar volume for conversion of ice to liquid water (0°C, 1 atm) equals to ΔV = −1.621 cm3.mol−1.[36] An increase in volume of water upon freezing means that with an increase in pressure the freezing point of water should be slightly smaller. Actually, it drops to −1°C at approximately 130 atmospheres.[40] This indicates that water has a high percentage of molecules involved in the transient and dynamic hydrogen bonding. When ice melts to the liquid water, the regulate structure collapses to some extent, but approximately 85% of the hydrogen bonds remain.[11,13] The liquid water at 0°C is only 15% less hydrogen bonded than ice. The percentage of hydrogen bonds in liquid water decreases as temperature increases. At boiling point (100°C, 1 atm) there is a large increase in the molar volume; i.e., its level rises to a value (3.014 × 104 cm3.mol−1) near the ideal gas (V = RT/V = 3.062 × 104).[13,43]
Volume of the liquid water at temperature T is calculated by assuming a linear relationship of expansion volume with temperature according to:(5)where β is the thermal coefficient of volume expansion, V(T 0) is the volume of liquid water at T 0 (273 K), and (T − T 0) is the temperature change. The thermal coefficient of volume expansion at T 0 (273 K) is given by:(6)
The thermal coefficient of water above 0°C is greater than that of metals and metal oxides. The molecular structure of water changes during the phase conversion by formation or breaking of hydrogen bonds. Upon solidification of water and formation of hydrogen bonds within the ice lattice, its electronic structure is modified. Consequently, small changes in the equilibrium positions of the protons, reorientation of hydrogen bonds with higher stability, take place. Alternatively, additional hydrogen bonds between the molecules are formed. The expansion of volume can be expressed as a function of the formation of H-bonds. The average hydrogen bond energy in ice was reported to be 28.1 kJ.mol−1, whereas it is 23.9 kJ.mol−1 for the liquid water.[6,10] The total energy needed to break all of hydrogen bonds in water at 100°C was estimated to be 30.6 kJ.mol−1. The structural change can be evaluated by comparing cohesive energies of ice Ih (48.9 kJ.mol−1) and that of liquid water (46.2 kJ.mol−1) at 0°C.
For many applications, it is satisfactory to treat the volume expansion, β, of solids and liquids as independent of pressure. It may usually be treated as constant even for pressure changes of the order of several hundred atmospheres. Occasionally their temperature dependence can be neglected. The temperature variation of β is more significant for water and ice in comparison with substances similar to water. The value of β is negative at very low temperatures and also in the region of 0–4°C.[44] The value of β is usually calculated from an empirical equation, which relates the density variation to temperature at constant pressure. Since the molar (or specific) volume, V, is reciprocal of the molar (or specific) density, ρ, the value of β is expressed as:(7)
In some experiments on the expansion of solid, linear expansion, α, has been measured rather than the volume expansion, β.[44]
Thermal Properties
Heat capacity
Another remarkable property of water is its extremely high capacity to absorb heat without a significant increase in temperature. The liquid water has the largest heat capacity among all liquids and solids except NH3,[16,45] and has the largest heat capacity per unit mass among all substances. Heat capacity of the liquid water at 0°C (76.1 J.mol−1.K−1) is approximately two times that of ice (37.3 J.mol−1.K−1).[7,9] This is because with a rise in temperature, the energy of the liquid water is consumed for both increase in thermal motion of water molecules, and for the rupture of the hydrogen bonds. There are many interactions between water molecules in the liquid state which are broken gradually as the temperature increases.[43] The heat capacity of water at the melting point is infinite, since heat is taken up with no change in temperature. At this temperature a large number of water molecules act together in order to change from one state to another one with a negligible change in temperature (cooperative transition).[43] One can calculate the difference between heat capacities at constant pressure and volume based on the following equation:(8)where CP and CV are heat capacities at constant pressure (isobaric) and constant volume (isochoric), respectively. At 4°C (more exact: 3.98°C), the value of equals to zero, thus, one can reach the following equation:[9](9)
Enthalpy of a liquid at a definite temperature can be determined either by experiment or by calculation based on the thermodynamic relationships, if the data on heat capacity is available. Depending on the properties of state, the heat capacity, CP , can either increase or decrease with temperature. The heat capacities of the liquid water at three different temperatures are given in Table 4.[7,9,46] The variation of heat capacity, CP , with temperature at different pressures was illustrated in Fig. 2.[9] The heat capacity has a minimum value around 20°C. In the figure, it can be seen between 0 and 50°C. The heat capacity increases significantly when the curve approaches the saturation line (see dotted line in Fig. 2). At a temperature of 20°C, the value of CP as a function of pressure was given in Table 5.[9] The difference in the value of CP at different pressures and the difference between CP and CV for the liquid water at different temperatures are small (Tables 4 and 5). The heat capacity of the liquid water changes slightly with an increase in pressure (Table 5). An increase in pressure from 0.1 to 100 MPa causes a change in heat capacity by 5%.
Figure 2 Heat capacity of water, CP, as a function of temperature at different pressures. (Reproduced from Kirillin, V.A.; Sychev, V.V.; Schindlin, A.E. Engineering Thermodynamic; Mir Publishers: Moscow, 1987.)
Heat capacity is a useful concept and finds application in heat transfer. The large heat capacity of water makes water as an excellent reservoir and transferer of energy. Water is used as a good coolant in condensers to keep foodstuffs cool from over-heating.[16] It is also widely used for heat transfer in food processing. To heat a kilogram of water from 20 to 50°C requires about 125 kJ of energy, whereas heating the same mass of vegetable oil requires only 44 kJ.[45] Heat transfer phenomenon involved in freezing of biological materials as well as foods is basically nonlinear.[30] Heat capacity can be also used to estimate the extent of hydrogen bonding in water.[41]
Boiling and freezing points
Water has unusually high boiling point (100°C) and freezing point (0°C) in comparison with hydrides of B, C, N, F, S, Se, and Te.[1–7] All of the latter hydrides are in gas state at atmospheric pressure. Water would be expected to have boiling and freezing points close to above-mentioned hydrides. Indeed, the boiling point of water is 264°C higher than that of methane, a substance with nearly the same molecular mass as H2O, but incapable of hydrogen bonds (in the absence of intermolecular association). Extrapolation of the plot of boiling points versus molecular weights gives an expected boiling point for water (−75°C).[47] NH3 and HF, which mutually hydrogen bonded, have much lower boiling points than that of water. Methanol has only one hydrogen donor site, and dimethyl ether has none, but has a hydrogen bond acceptor site, whereas water forms a structural network.[4] This difference reflects the extraordinary internal cohesiveness of liquid water resulting from its intermolecular hydrogen bonding.[11,41]
Latent heats of fusion, evaporation, and sub-limitation
The latent heats of fusion (6.01 kJ.mol−1), evaporation (40.6 kJ.mol−1), and sublimation (51.2 kJ.mol−1) of water are high value.[1–7] The high values are due to both electrostatic and van der Waals forces between the water molecules in the liquid and solid states. The latent heat of water represents a large amount of heat that has to be removed from the foodstuffs or has to be given them.[30] The latent heat of evaporation (40.6 kJ.mol−1) is more than six times greater than that of the amount of heat required to raise water temperature from freezing to boiling point. The high value of heat of evaporation is due to breaking of all hydrogen bonding at 100°C. The latent heat of evaporation can be used to estimate the energy of hydrogen bonds in water. Based on comparison with data for similar substances, the latent heat of evaporation of non-hydrogen-bonded H2O should be 10.1 kJ.mol−1, about one-fourth of the actual value (40.6 kJ.mol−1), and one-fifth of the heat of evaporation methane. The difference is ascribed to the energy of the hydrogen bonds. Removing water from foods by sublimation or by freeze drying process is associated with withdrawing or vaporization of water molecules.
Thermal conductivity
The thermal conductivity of water is large in comparison with other liquids.[2] The thermal conductivity of ice is moderately large compared to that of other non-metallic solids. The thermal conductivity of ice at 0°C is approximately four times greater than that of the liquid water at the same temperature (Tables 1 and 2), indicating that ice will conduct heat energy much faster than immobilized water, e.g., in tissue.[2] Thermal conductivity of most liquids decreases linearly with an increase in temperature. In contrast, water shows an anomalous increase in thermal conductivity with an increase in temperature (Table 4).[7,9]
VISCOSITY
Viscosity and thermal conductivity are two of key transport properties. Dynamic viscosity (measure of internal fluid friction, i.e., resistance to deformation) of water is greater than that of comparable substances without hydrogen bonds. The viscosity drops dramatically with an increase in temperature (Table 4). The dependence can be described by a conventional Arhenius-type expression.[46]
Pure water and aqueuos solutions containing low-molecular weight solutes such as salts and sugars under normal conditions are Newtonian fluids (shear stress is directly proportional to rate of deformation or rate of shear strain). There is a rise in viscosity when a nonvolatile substance is dissolved in water.[3] In general, aqueous solutions contain large-molecular weight food components (proteins, polysaccharides, hyrocolloids), even though only small amounts are non-Newtonian.
SURFACE TENSION
With the exception of mercury, water has the highest surface tension of any other liquids. Polar compounds tend to have much higher surface tension than non-polar compounds. The surface tension of water (72.8 mN.m−1 or dynes per cm at 20°C) is much higher than that of ethyl alcohol (24 dynes per cm).[46] Hydrogen bonding of water molecules is responsible for its high surface tension value. Hydrogen bonding is attributed to the ability of water to adhere to or wet to most surfaces (hydrophilic substances). Other substances, such as oils, fats, waxes, and many synthetics polymers (hydrophobic substances), will not wet with water. There is a correlation between surface hydrophobicity as well as hydrophilicity of a food component, such as a protein and its ability to change surface and interfacial tensions.[2] Surface tension of water decreases with an increase in temperature (Table 4); the decrease is nearly linear as a function of absolute temperature. Surface tension at the critical temperature of water is zero.[46] In the food industry, dioctyl sodium sulfosuccinate and disodium hydrogen phosphate as surface tension control agents have been used.[2]
SOLUBILITY OF MATERIALS IN WATER
Water is a universal solvent as well as a solvent for many substances because of strong interactions between water and other substances, its high dipole moment, and its ability to donate and accept protons for formation of hydrogen bonds. These properties make it an excellent solvent, especially for electrolytes and polar compounds (salts, acids, sugars, alcohols, carbonyl compounds, etc.).[8,10,11,47,48] More than half of known elements in the periodic table can be found in natural water, some in high concentrations, and others only in trace amounts. Water has been called a green solvent. It is a safe solvent and has advantages over polluting organic solvents due to the environmental concerns.
The thermodynamic state (it is expressed by its Gibbs free energy, which is a criterion of feasibility of chemical or physical transformation) of water in food arises first from unusual properties of water and its ability to form strong hydrogen bonds.[49] Porosity of the materials, which is quite common for foods, also affects the thermodynamic state of water.[49] Interactions with hydrophilic solute lead to the formation of hydrated components. On the other hand, interactions with hydrophobic solute affect the structure of the solvent water.[49] The dissolution of some food components such as sugars in water is because of bonding between water molecule and the polar groups of the food components. The dissolution of salts is due to electrostatic forces between water and ions created from the salts. The water molecule greatly weakens electrostatic forces and hydrogen bonds between polar molecules by competing with their attraction forces. The later forces are greater than that of the attraction forces between ions of salts.
Water is used as a solvent or diluents in preparation of beverages and foodstuffs. A foodstuff ususally contains various organic and inorganic substances. These solutes modify the properties of water. At ambient temperature and pressure, the food components (carbohydrates, proteins, minerals, acids) are miscible in water, while lipids have fairly little interactions with water.
Water is classified as soft, medium and hard. Water hardness is classified based on the amounts of removable calcium carbonate salt per unit volume from water. Water hardness is a critical factor in food processing. It can dramatically affect the quality of food products. The hardness of water may be altered or treated by using a chemical ion exchange system.[47,48] The hardness of water is influenced by pH of medium, which plays a critical role in food processing. For example, hard water prevents successful production of clear beverages.
The physical properties of water (e.g., boiling and freezing points) are affected by solutes such as salts and sugars in water, beverages, and foodstuffs. One mole of sucrose per kilogram of water raises the boiling point of water by 0.51°C, and one mole of sodium chloride per kilogram raises the boiling point by 1.02°C. Similarly, increasing the number of dissolved particles reduces the freezing point of water.[2–5] For example, it is −8.4 and −16°C for 7% solutions of K2SO4 and NaCl, respectively.[8,13,48] Freezing point depression of water is related to water activity.[50] Freezing point depression is used to check for adulterations of milk.[50] This property of water can be also used to verify for adulteration of other beverages and foods. Adsorption, extraction, filtration, and osmotic procedures can be employed to remove several contaminants from both ground and surface water. Drinking water may also be prepared when salty water freezes. The drinking water produced from the latter procedure is almost pure, and it contains only very little salts.
WATER CONTENT AND WATER ACTIVITY
Water, as a constituent of food, may affect food safety, stability, quality, and physical properties. Range of water concentration (or water content: the amount of water in a food per amount of its dried food) in food is very broad and begins with a fraction of a percent and reaches even more than 98%. Fresh and liquid foods contain usually large amounts of water while baked and dried foods are poor in water content.[8,51,52] In food products with high water content, such as fresh foods, water loss alters physical characteristics and water gain leads to favorable conditions for microbiological growth. Thus, food packaging is performed against gain or loss of water.
Water activity, aw , is described as a ratio of the vapor pressure of water in a solution to the vapor pressure of pure water. Water activity, at any temperature and water content, can be measured by determining the equilibrium relative humidity (ERH) surrounding the food in a sealed container. It is a measure of free moisture or available water in a food product.[2–5,49,51] Water activity is used to estimate whether a foodstuff absorbs or loses water in a given environment. It is a critical parameter to evaluate food quality.[2–5] The water activity in a solution or a foodstuff is derived from its chemical potential. The chemical potential of pure water is given by its molar Gibbs-free energy.[2–5,15] The water activity reflects combined effects of water-solute, water-surface, capillary, hydrophilic, and hydrophobic interactions.
Many food properties correlate better with water activity than with water content, especially if the food contains substances that are more or less indifferent to water, like crystals or oil droplets. This does not mean that relations between a property and water activity are the same for all foods, not even in a relative sense. Some properties, notability rheological ones, generally correlate better with water content than with water activity.[53,54] Thus, knowledge on water content is not enough information to evaluate food safety and stability during storage.
Water content as a function of vapor pressure at equilibrium (P/P 0), and at a constant temperature, is designated a sorption isotherm. Water tends to be adsorbed in the form of clusters. In general, a monolayer of water (Brunauer-Emmert-Teller, BET) is critical water content, below which dehydrated foods are stable. The BET isotherm is applicable at low water activity, aw .[2] A plot of moisture content versus water activity at any temperature (moisture-sorption isotherm) can be used: (i) to optimize formulation, concentration, and dehydration processes; (ii) to optimize packaging conditions and to control microbial growth; and (iii) to control physical and chemical stability of food products.[6] When the value of aw is low, foods (carbohydrates, lipids, or proteins) behave more like rubbery polymers than crystalline structures. The water substance may be physically immobilized as a glassy material, it may be bound to food components (proteins or carbohydrates) in food systems with very low aw or it may be bound with food components under freezing conditions. Under the latter conditions, the water molecule may diffuse with difficulty and thus may inhibit the diffusion of solutes. Changes in the structure of carbohydrates and proteins from amorphous to crystalline or vice versa may also take place only very slowly by water migration or diffusion.[55] Knowledge on water sorption behavior, and its relation to glass transition temperature, Tg (from a rubbery or glassy structure to a more crystalline one) provides a valuable tool: (a) to control material behavior during processing and subsequent storage; and (b) to determine food-packaging requirements.[55] The Tg is an important parameter for long-term storage stability of frozen foods (for example ice creams), and controlling textural properties of cookies and chips.[6] For baked or dried foods, which are stored at room temperature, the Tg may indicate a temperature at which staling, loss of softness, or the development of an undesirable texture may take place.[6,56]
Water activity of solutions and liquid foods depends on concentration, chemical nature of solute and temperature. Water activity of fresh foods (meats, sea foods, most dairy products, fresh fruits, and vegetable) is high and is close to 1.[49] Furthermore, high-fat products with relatively small amounts of water, such as butter and margarine, have a relatively high water activity. When water activity is smaller than 0.9, most molds are inhibited. Most of microorganisms cease growing if water activity is smaller than 0.6.[25,56,57] The reduction of water activity retards the growth of micro-organisms, non-enzymatic browning, and enzymatic reactions.
The rate of water adsorption or desorption of foods depends on their physical states and temperature. The major physical change that occurs during storage of frozen food results from water migration. The water migration causes water content changes or changes in ice crystal size called re-crystallization. Water migration can correspond either to a water loss through surface dehydration or to a water location change for products with heterogeneous water contents.[6,58]
Water activity is a vital parameter, because it affects texture, taste, appearance, safety, and shelf life of foods. Water activity is an important factor in concentrated foods and dehydrated foods or foods those contain salts, and sugars.[59] It is often of great interest in food product development to estimate effect of food composition on water activity of end-products. It is also important in packaging of foodstuffs with different ingredients having different water activities. A foodstuff containing several components with different water activities are time-dependent for water migration until equilibrium is attained.[59,60] The level of water loss and the rate of deterioration depend strongly on water content and water activity.
REACTION OF WATER WITH OTHER COMPONENTS OF FOOD
Several reactions, such as chemical or biochemical hydrolysis (lipids, carbohydrates, and proteins) and enzymatic and non-enzymatic (Maillard) browning, occur in the presence of water. Oxidative reaction is a common reaction in low-moisture foods. Water can be a reducing or oxidizing reagent, because it offers protons or electrons. Oxidation-reduction reactions involving water usually is due to proton or electron transfer. The oxidation-reduction reactions of water cause corrosion on metal surfaces. The latter reactions (electrochemical processes) in food industry result in corrosion in processing lines and reaction vessels, and produce metal ions (Cu2+, Pb2+, and Fe+2) in drinking water, beverages, and foodstuffs.[5] The presence of some metal ions (Cu2+, Pb2+, Fe+2, Sn+2) as residues in drinking water, beverages, and canned foods result in unsafe and off-flavor products.
The reactions of food components as well as rates of most reactions in food chains are affected by water activity. The stability of foods, rates of several deteriorative reactions (enzymatic and non-enzymatic browning, oxidative reactions, chemical or biochemical hydrolysis, enzyme-catalyzed flavor changes, chlorophyll degradation, and anthocyanin degradation), and microbial growth depends on water activity, water content, and state of water. The rates of both enzymatic and non-enzymatic reactions increase as water activity increases. The deterioration of vitamin C increases rapidly as the water activity increases. Rates of almost all reactions increase as water content increases over the lower portion of a multilayer zone. Addition of water beyond boundary of mono-layers and multilayer zones results in an increase in rates of oxidation. Karel and Yong[61] have suggested that water added in this region of the isotherm may accelerate oxidation by increasing the solubility of oxygen and by allowing macromolecules to swell, thereby exposing more catalytic sites. In dried and fat-containing foods with very low moisture contents (aw < 0.1) oxidation proceeds very rapidly. At water activity smaller or equal to 0.3, lipid oxidation retards by reducing catalytic activity of metal catalysts, promoting non-enzymatic browning, and/or by impeding the access of oxygen to foods. At higher water activities (aw = 0.55–0.80), oxidation rate increases by increasing mobilization of catalysts.[2] At water activities more than 0.80, the added water may retard rates of oxidation, and dilution of catalysts reduces their effectiveness.[2,61]
The rates of non-enzymatic browning (Maillard reaction) are greatest in foods with an intermediate moisture contents (bakery products, roasted nuts, toasted breakfast cereals, and roller-dried milk powder).[2] Undesirable Maillard reaction can be inhibited by decreasing moisture to a very low level or by increasing dilution (if the food is in liquid state).
Reaction rates are influenced by concentration of solutes.[2,47,58] Removal of water from foods in the process of concentration, dehydration, and freezing of foods reduce the rate of deterioration (microbial and enzymatic).[57,62] A decrease in temperature generally decreases the rate of chemical and biochemical reactions. Deteriorative reactions are slowed down at 0°C and at normal temperature of frozen storage (−18°C).[47,59]
GLOBAL VIEW ON WATER STRUCTURE-WATER PROPERTIES-FOOD APPLICATIONS RELATIONSHIPS
The properties of water in comparison with other substances and their importance to food technology are given in Table 6. Many operations in food processing use physicochemical properties of water and its state transition properties. The transition of water from liquid into solid plays an important role in storage and packaging of foods. Water is a reactant for several reactions occuring in food systems. Rheological and transport properties of food systems depend strongly on water content and the quality of interactions between water and other food components. In the food industry, water is used as an excellent medium for heating and cooling operations. The unusual thermal properties of water make it a suitable environment for biological systems as well as an excellent medium for chemical processes of food systems.
CONCLUSIONS
Water is an essential component of foods. It combines with food components (protein, carbohydrates, phospholipids, water-soluble vitamins, etc.) by means of polar, hydrogen bonding, hydrophilic, and hydrophobic interactions. Among the various types of intermolecular interactions, the hydrogen bond plays the most significant role in determining the structure and properties of water and food components. A high value for dipole moment and contribution of hydrogen bonds in water associations are responsible for its several unusual properties. Free energy of cohesion for polar and van der Waals contributions represent 70 and 30% of the total energy for the liquid water, respectively. The effect of hydrogen bond formation is central to understanding unusual properties of water. The structure and properties of water change based on its state, temperature, and pressure. The properties of water in foods are different from those of pure water. Knowledge on the properties of pure water is a prerequisite for understanding their effects on other individual food components and combined foodstuffs. The quality and cost of foodstuffs depends strongly on their water content and water activity. Water plays important roles in many technical processes and its properties are used in a wide range of food technologies. The rate of chemical and biochemical reactions can be controlled by water activity, pH, and temperature. Storage, shelf-life, stability, and microbial safety of foodstuffs depend on their water content and water activity.
REFERENCES
Lerner, K.L. and Lerner, B.W. 2005. “Basics of water science”. In Encyclopedia of Water Science Vol. 3, 1–22. Thomson Gale, New York [Google Scholar]
Fennema, O.R. 1996. Food Chemistry, 3rd, New York: Marcel Dekker, Inc. [Google Scholar]
Alais, C. and Linden, G. 1991. Food Biochemistry Edited by: Morton, I. and Whitehead, A. 95–103. Ellis Horwood, New York Translated from French to English by [Crossref], [Google Scholar]
Belitz, H.-D., Grosch, W. and Schieberle, P. 2009. Food Chemistry, Berlin: Springer-Verlag. [Google Scholar]
de Man, J.M. 1999. Principles of Food Chemistry, 3rd, Gaithersburg, MD: Aspen Publishers Inc.. [Crossref], [Google Scholar]
Hardly, J., Scher, J. and Banon, S. 2002. Water activity and hydration of dairy powders. Lait, 82: 411–452. [Google Scholar]
Lide, D.K. CRC Handbook of Chemistry and Physics, 83rd, 2002–2003. Boca Raton, FL: CRC Press. [Google Scholar]
Lewicki, P.P. 2004. Water as the determinant of food engineering properties. A review. Journal of Food Engineering, 61: 483–495. [Crossref], [Web of Science ®], [Google Scholar]
Kirillin, V.A., Sychev, V.V. and Schindlin, A.E. 1987. Engineering Thermodynamic, Mir Publishers: Moscow. [Google Scholar]
Engberts, J.B.F.N. 2007. “Organic Reactions in Water: Principles, Strategies and Applications”. In Structure and properties of water, 29–59. Oxford, UK: Blackwell Publishing. [Google Scholar]
Franks, F. 2000. Water: A Matrix of Life, 2nd, Cambridge, UK: Royal Society of Chemistry. [Google Scholar]
van Oss, C.J. 2008. The Properties of Water and Their Role in Colloidal and Biological Systems; Elsevier: Amsterdam 1–9. [Crossref], [Google Scholar]
Chieh, C. 2006. “Fundamental characteristics of water”. In Handbook of Food Science, Technology, and Engineering, Edited by: Hui, Y.H. Vol. 1, 12-1–12-17. Boca Raton, FL: Taylor & Francis. [Google Scholar]
Dahl, L.W. and Andersen, H.C. 1980. A theory of the anomalous thermodynamic properties of liquid water. Journal of Chemical Physics, 78(4): 1980–1993. [Crossref], [Web of Science ®], [Google Scholar]
Franks, F. Water. and Coultate, T.P. 2002. Food: The Chemistry of its Components , 4th, 389–408. London: The Royal Society of Chemistry, Paperbacks. [Google Scholar]
Atkins, P.W. 1988. Physical Chemistry, 136–159. Oxford University Press: Oxford. [Google Scholar]
Suresh, S.J. and Naik, V.M. 2000. Hydrogen bond thermodynamic properties of water from dielectric constant data. Journal of Chemical Physics, 111: 9727–9732. [Crossref], [Web of Science ®], [Google Scholar]
Chaplin, M. Water structure and behavior. http://www.lsbu.ac.uk/water/ (http://www.lsbu.ac.uk/water/) [Google Scholar]
Kasaai, M.R. 2008. A review of several reported procedures to determine the degree of N-acetylation for chitin and chitosan using infrared spectroscopy. Carbohydrate Polymers, 71: 497–508. [Crossref], [Web of Science ®], [Google Scholar]
Gun'ko, V.M., Turov, V.V., Bogatyrev, V.M., Zarko, V.I., Leboda, R., Gonchruk, E.V., Novza, A.A., Turov, A.V. and Chuiko, A.A. 2005. Unusual properties of water at hydrophilic/hydrophobic interfaces. Advances in Colloid Interface Science, 118: 125–172. [PubMed], [Web of Science ®], [Google Scholar]
Kasaai, M.R. 2010. Determination of the degree of N-acetylation for chitin and chitosan by various NMR spectroscopy techniques: A review. Carbohydrate Polymers, 79: 801–808. [Crossref], [Web of Science ®], [Google Scholar]
Busk, G.C. May 1984. Polymer-water interactions in gelation, May, 59–64. Food Technology. [Google Scholar]
Sammon, S., Deng, C. and Yarwood, J. 2003. Polymer. Polymer-water interactions. Origin of perturbed infrared intensities of water in polymeric systems, 44: 2669–2677. [Google Scholar]
Hatakeyama, H. and Hatakeyama, T. 1998. Interaction between water and hydrophilic polymers. Thermochimica Acta, 308: 3–22. [Crossref], [Web of Science ®], [Google Scholar]
Labuza, T.P. 1977. The properties of water in relationship to water binding in foods: A review. Journal of Food Processing and Preservation, 1(2): 167–190. [Crossref], [Google Scholar]
Gun'ko, V.M. and Turov, V.V. 1999. Structure of hydrogen bonds and 1H NMR spectra of water at the interface of oxides, Vol. 15, 6405Langmuir. [Google Scholar]
Li, J. and Ross, D.K. 1993. Evidence for two kinds of hydrogen bond in ice. Nature, 365: 327–329. [Crossref], [Web of Science ®], [Google Scholar]
Jenkins, S. and Morrison, I. 1999. Characterization of various phases of ice on the basis of the charge density. Journal of Physical Chemistry, Part B, 103: 11041–11049. [Crossref], [Web of Science ®], [Google Scholar]
Wiggins, P. Life dependence upon two kinds of water. 2008. PloS One. http://www.plosone.org (http://www.plosone.org) (Accessed: 1 January 2008). [Google Scholar]
Bail, A.L., Hui, Y.H., Cornillon, P., Legeretta, I.G., Lim, M.H., Murrell, K.D. and Nip, W.-K. 2004. “Freezing processes: Physical aspects”. In Handbook of Frozen Foods, 1–11. New York: Marcel Dekker Inc.. [Google Scholar]
Jiang, S.-T., Lee, T.-C., Hui, Y.H., Cornillon, P., Legeretta, I.G., Lim, M.H., Murrell, K.D. and Nip, W.-K. 2004. “Freezing seafood and seafood products: Principles and applications”. In Handbook of Frozen Foods, 245–294. New York: Marcel Dekker, Inc.. [Crossref], [Google Scholar]
Welti-Chanes, J., Bermúdez, D., Valdez-Fragosa, A., Mújica-Paz, H., Alzamora, S.M., Hui, Y.H., Cornillon, P., Legeretta, I.G., Lim, M.H., Murrell, K.D. and Nip, W.-K. 2004. “Principles of freeze-concentration and freeze-drying”. In In: Handbook of Frozen Foods, Eds, 13–24. New York: Marcel Dekker, Inc.. [Google Scholar]
Liu, W.G. and Yao, K.D. 2001. What causes the unfrozen water in polymers: hydrogen bonds between water and polymer chains? Polymer, 42: 3943–3947. [Google Scholar]
Edsall, J.T. and Makenzie, H.A. 1983. Water and proteins. II. The locations and dynamics of water in protein systems and its relations to their stability and properties. Advances in Biophysics, 16: 53–183. [Crossref], [PubMed], [Web of Science ®], [Google Scholar]
Hoffman, A.S., Afrasiabi, A. and Dong, L.C. 1986. Thermally reversible hydrogels: Delivery and selective hydro-gels. Journal of Controlled Release, 4: 213–222. [Crossref], [Google Scholar]
Gallier, J., Rivet, P. and de Certaines, J.D. 1987. 1H- and 2H NMR study of bovine serum albumin solutions. Biochimie Biophysic Acta, 915: 1–18. [Crossref], [PubMed], [Web of Science ®], [Google Scholar]
Crosby, N.T. 1981. Food Packaging Materials; Applied Science Publishers, Ltd.: London,. [Google Scholar]
Coles, R., McDowell, D. and Kirwan, M.J. 2003. Food Packaging Technology; CRC Press: Boca Raton FL [Google Scholar]
Mathlouthi, M. 1986. Food Packaging and Preservation. Theory and Practice; Elsevier Applied Science Publishers: London [Google Scholar]
Kireev, V. 1979. Physical Chemistry; Mir Publishers: Moscow 144–148. [Google Scholar]
Eisenberg, D. and Kauzmann, W. The Structure and Properties of Water, Oxford. 1969: Oxford University Press. [Google Scholar]
Makkonen, L. 1997. Surface melting of ice. Journal of Physical Chemistry, Part B, 101: 6196–6200. [Crossref], [Web of Science ®], [Google Scholar]
Eisenberg, D. and Crothers, D. 1979. “Physical Chemistry with Applications to the Life Science”. In The Benjamin/ Cumming Publishing Company, Inc, 68–75. Amsterdam. [Google Scholar]
Zemansky, M.W., Abbott, M.M. and Van Ness, H.C. 1987. “Basic Engineering Thermodynamics”. In Rensselaer Polytechnic, Institute: New York. [Google Scholar]
Cybulska, B., Doe, P.E. and Sikoroski, Z.E. 2002. “Chemical and Functional Properties of Food Components”. In Water and food quality, Ed, 25–50. Boca Raton, FL: CRC Press. [Google Scholar]
Fox, R.W. and McDonald, A.T. 1998. Introduction to Fluid Mechanics, 112–133. New York: John Wiley & Sons, Inc. [Google Scholar]
Eubanks, L.P., Middlecamp, C.H., Pienta, N.J., Heltzel, C.E. and Weaver, G.C. 2006. Chemistry in Context: Applying Chemistry to Society, 219–265. Boston: McGraw Hill. [Google Scholar]
Stango, M. 2010. “Sanitation: Cleaning and Disinfection in the Food Industry”. In Wiley-VCH: Darmstadt 1–75. Germany [Google Scholar]
Lewicki, P.P. 2009. “Data and models of water activity. 1: Solutions and liquid foods. In”. In Food Properties Handbook, 2nd, 33–65. Boca Raton, FL: CRC Press. [Google Scholar]
Rockland, L.B. and Beuchat, L.R. 1987. Water Activity: Theory and Applications to Food; Marcel Dekker, Inc.: New York [Google Scholar]
Lewicki, P.P. 2009. “Data and models of water activity. II: Solid foods. In”. In Food Properties Handbook, 2nd, 67–151. Boca Raton FL: CRC Press. [Google Scholar]
Rahman, M.S. and Sahlani, S.S. 2009. “Water activity measurement methods of foods. In”. In Food Properties Handbook, 2nd, 9–32. Boca Raton, FL: CRC Press. [Crossref], [Google Scholar]
Walestra, P. 2003. Physical Chemistry of Foods, New York: Marcel Dekker. [Google Scholar]
Isengard, H-D. 2001. Water content, one of the most important properties of food. Food Control, 12: 395–400. [Crossref], [Web of Science ®], [Google Scholar]
Slade, L. and Lavine, H. 1991. Beyond water activity: Recent advances based on an alternative approach to the assessment of food quality and safety, 115–360. FL: Critical Review in Food Science and. Nutrition; CRC Press: Boca Raton. [Google Scholar]
Roos, Y.H. 2002. Importance of glass transition and water activity to spray drying and stability of dairy powders. Lait, 82: 475–484. [Crossref], [Google Scholar]
Chen, X.D. 2010. “Processing Effects on Safety and Quality of Foods”. In Drying and dried food quality, 323–340. CRC Press: Boca Raton, FL: Ortega-Rivas, E.. [Google Scholar]
Blond, G., Meste, M.L., Hui, Y.H., Cornillon, P., Legeretta, I.G., Lim, M.H., Murrell, K.D. and Nip, W.-K. 2004. “Principles of frozen storage”. In Handbook of Frozen Foods, 25–53. New York: Marcel Dekker, Inc. [Google Scholar]
Roos, Y.H. 1993. Water activity and physical state effects on amorphous food stability. Journal of Food Processing and Preservation, 16: 433–447. [Crossref], [Web of Science ®], [Google Scholar]
Roos, Y.H., Eskin, N.A.M. and Robinson, D.S. 2000. “Water activity and plasticization”. In Food Shelf Life Stability;, Eds, Boca Raton, FL: CRC Press. [Google Scholar]
Karel, M., Yong, S., Rockland, L.B. and Steward, G.F. 1981. “Autoxidation-initiated reactions in food”. In Water Activity, Influence on Food Quality, Eds, 511–529. New York: Academic Press. [Crossref], [Google Scholar]
Hui, Y.H., Cornillon, P., Legeretta, I.G., Lim, M.H., Murrell, K.D. and Nip, W.-K. 2004. Handbook of Frozen Foods, Eds, New York: Marcel Dekker, Inc. [Google Scholar]
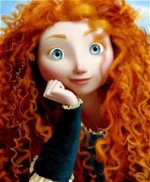