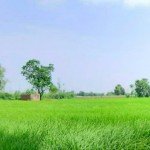
Abstract
The epidemic nature of diabetes mellitus in different regions is reviewed. The Middle East and North Africa region has the highest prevalence of diabetes in adults (10.9%) whereas, the Western Pacific region has the highest number of adults diagnosed with diabetes and has countries with the highest prevalence of diabetes (37.5%). Different classes of diabetes mellitus, type 1, type 2, gestational diabetes and other types of diabetes mellitus are compared in terms of diagnostic criteria, etiology and genetics. The molecular genetics of diabetes received extensive attention in recent years by many prominent investigators and research groups in the biomedical field. A large array of mutations and single nucleotide polymorphisms in genes that play a role in the various steps and pathways involved in glucose metabolism and the development, control and function of pancreatic cells at various levels are reviewed. The major advances in the molecular understanding of diabetes in relation to the different types of diabetes in comparison to the previous understanding in this field are briefly reviewed here. Despite the accumulation of extensive data at the molecular and cellular levels, the mechanism of diabetes development and complications are still not fully understood. Definitely, more extensive research is needed in this field that will eventually reflect on the ultimate objective to improve diagnoses, therapy and minimize the chance of chronic complications development.
Keywords: Diabetes, Classification of diabetes, Type 1 diabetes, Type 2 diabetes, Gestational diabetes, Diagnosis, Etiology, Genetics
Core tip: Diabetes mellitus is rising to an alarming epidemic level. Early diagnosis of diabetes and prediabetes is essential using recommended hemoglobin A1c criteria for different types except for gestational diabetes. Screening for diabetes especially in underdeveloped countries is essential to reduce late diagnosis. Diabetes development involves the interaction between genetic and non-genetic factors. Biomedical research continues to provide new insights in our understanding of the mechanism of diabetes development that is reviewed here. Recent studies may provide tools for the use of several genes as targets for risk assessment, therapeutic strategies and prediction of complications.
DEFINITION OF DIABETES MELLITUS
Diabetes mellitus is a group of metabolic diseases characterized by chronic hyperglycemia resulting from defects in insulin secretion, insulin action, or both. Metabolic abnormalities in carbohydrates, lipids, and proteins result from the importance of insulin as an anabolic hormone. Low levels of insulin to achieve adequate response and/or insulin resistance of target tissues, mainly skeletal muscles, adipose tissue, and to a lesser extent, liver, at the level of insulin receptors, signal transduction system, and/or effector enzymes or genes are responsible for these metabolic abnormalities. The severity of symptoms is due to the type and duration of diabetes. Some of the diabetes patients are asymptomatic especially those with type 2 diabetes during the early years of the disease, others with marked hyperglycemia and especially in children with absolute insulin deficiency may suffer from polyuria, polydipsia, polyphagia, weight loss, and blurred vision. Uncontrolled diabetes may lead to stupor, coma and if not treated death, due to ketoacidosis or rare from nonketotic hyperosmolar syndrome[1-3].
CLASSIFICATION OF DIABETES MELLITUS
Although classification of diabetes is important and has implications for the treatment strategies, this is not an easy task and many patients do not easily fit into a single class especially younger adults[1,4-6] and 10% of those initially classified may require revision[7]. The classical classification of diabetes as proposed by the American Diabetes Association (ADA) in 1997 as type 1, type 2, other types, and gestational diabetes mellitus (GDM) is still the most accepted classification and adopted by ADA[1]. Wilkin[8] proposed the accelerator hypothesis that argues “type 1 and type 2 diabetes are the same disorder of insulin resistance set against different genetic backgrounds”[9]. The difference between the two types relies on the tempo, the faster tempo reflecting the more susceptible genotype and earlier presentation in which obesity, and therefore, insulin resistance, is the center of the hypothesis. Other predictors of type 1 diabetes include increased height growth velocity[10,11] and impaired glucose sensitivity of β cells[12]. The implications of increased free radicals, oxidative stress, and many metabolic stressors in the development, pathogenesis and complications of diabetes mellitus[13-18] are very strong and well documented despite the inconsistency of the clinical trials using antioxidants in the treatment regimens of diabetes[19-21]. The female hormone 17-β estradiol acting through the estrogen receptor-α (ER-α) is essential for the development and preservation of pancreatic β cell function since it was clearly demonstrated that induced oxidative stress leads to β-cell destruction in ER-α knockout mouse. The ER-α receptor activity protects pancreatic islets against glucolipotoxicity and therefore prevents β-cell dysfunction[22].
TYPE 1 DIABETES MELLITUS
Autoimmune type 1 diabetes
This type of diabetes constitutes 5%-10% of subjects diagnosed with diabetes[23] and is due to destruction of β cells of the pancreas[24,25]. Type 1 diabetes accounts for 80%-90% of diabetes in children and adolescents[2,26]. According to International Diabetes Federation (IDF), the number of youth (0-14 years) diagnosed with type 1 diabetes worldwide in 2013 was 497100 (Table (Table1)1) and the number of newly diagnosed cases per year was 78900[27]. These figures do not represent the total number of type 1 diabetes patients because of the high prevalence of type 1 diabetes in adolescence and adults above 14 years of age. One reported estimate of type 1 diabetes in the United States in 2010 was 3 million[28,29]. The number of youth in the United States younger than 20 years with type 1 diabetes was estimated to be 166984 in the year 2009[30]. The prevalence of type 1 diabetes in the world is not known but in the United States in youth younger than 20 years was 1.93 per 1000 in 2009 (0.35-2.55 in different ethnic groups) with 2.6%-2.7% relative annual increase[26,31]. Type 1 diabetes is mainly due to an autoimmune destruction of the pancreatic β cells through T-cell mediated inflammatory response (insulitis) as well as a humoral (B cell) response[25]. The presence of autoantibodies against the pancreatic islet cells is the hallmark of type 1 diabetes, even though the role of these antibodies in the pathogenesis of the disease is not clear. These autoantibodies include islet cell autoantibodies, and autoantibodies to insulin (IAA), glutamic acid decarboxylase (GAD, GAD65), protein tyrosine phosphatase (IA2 and IA2β) and zinc transporter protein (ZnT8A)[32]. These pancreatic autoantibodies are characteristics of type 1 diabetes and could be detected in the serum of these patients months or years before the onset of the disease[33]. Autoimmune type 1 diabetes has strong HLA associations, with linkage to DR and DQ genes. HLA-DR/DQ alleles can be either predisposing or protective[1]. This autoimmune type 1 diabetes is characterized by the absence of insulin secretion and is more dominant in children and adolescents.
Number of subjects with type 1 diabetes in children (0-14 years), with diabetes in adults (20-79 years) and with hyperglycemia (type 2 or gestational diabetes) in pregnancy (20-49 years)
In addition to the importance of genetic predisposition in type 1 diabetes, several environmental factors have been implicated in the etiology of the disease[9,33]. Viral factors include congenital rubella[34,35], viral infection with enterovirus, rotavirus, herpes virus, cytomegalovirus, endogenous retrovirus[36,37] and Ljungan virus. Other factors include low vitamin D levels[38], prenatal exposure to pollutants, improved hygiene and living conditions decreased childhood infections in countries with high socioeconomic status leading to increased autoimmune diseases (hygiene hypothesis), early infant nutrition such as using cow’s milk formula instead of breast feeding[39] in addition to insulin resistance in early childhood due to obesity or increased height growth velocity. The role of environmental factors remains controversial[40]. Recent evidence supported the causative effect of viral infections in diabetes[41-43].
Type 1 diabetes often develops suddenly and can produce symptoms such as polydipsia, polyuria, enuresis, lack of energy, extreme tiredness, polyphagia, sudden weight loss, slow-healing wounds, recurrent infections and blurred vision[27] with severe dehydration and diabetic ketoacidosis in children and adolescents. The symptoms are more severe in children compared to adults. These autoimmune type 1 diabetes patients are also prone to other autoimmune disorders such as Graves’ disease, Hashimoto’s thyroiditis, Addison’s disease, vitiligo, celiac sprue, autoimmune hepatitis, myasthenia gravis, and pernicious anemia[1]. The complete dependence on insulin of type 1 diabetes patients may be interrupted by a honeymoon phase which lasts weeks to months or in some cases 2-3 years. In some children, the requirement for insulin therapy may drop to a point where insulin therapy could be withdrawn temporarily without detectable hyperglycemia[44].
Idiopathic type 1 diabetes
A rare form of type 1 diabetes of unknown origin (idiopathic), less severe than autoimmune type 1 diabetes and is not due to autoimmunity has been reported. Most patients with this type are of African or Asian descent and suffer from varying degrees of insulin deficiency and episodic ketoacidosis[45].
Fulminant type 1 diabetes
This is a distinct form of type 1 diabetes, first described in the year 2000, and has some common features with idiopathic type 1 diabetes being non-immune mediated[46,47]. It is characterized by ketoacidosis soon after the onset of hyperglycemia, high glucose levels (≥ 288 mg/dL) with undetectable levels of serum C-peptide, an indicator of endogenous insulin secretion[48]. It has been described mainly in East Asian countries and accounted for approximately 20% of acute-onset type 1 diabetes patients in Japan (5000-7000 cases) with an extremely rapid and almost complete beta-cell destruction resulting in nearly no residual insulin secretion[48,49]. Both genetic and environmental factors, especially viral infection, have been implicated in the disease. Anti-viral immune response may trigger the destruction of pancreatic beta cells through the accelerated immune reaction with no detectable autoantibodies against pancreatic beta cells[48,50]. Association of fulminant type 1 diabetes with pregnancy has also been reported[51].
TYPE 2 DIABETES MELLITUS
The global prevalence of diabetes in adults (20-79 years old) according to a report published in 2013 by the IDF was 8.3% (382 million people), with 14 million more men than women (198 million men vs 184 million women), the majority between the ages 40 and 59 years and the number is expected to rise beyond 592 million by 2035 with a 10.1% global prevalence. With 175 million cases still undiagnosed, the number of people currently suffering from diabetes exceeds half a billion. An additional 21 million women are diagnosed with hyperglycemia during pregnancy. The Middle East and North Africa region has the highest prevalence of diabetes (10.9%), however, Western Pacific region has the highest number of adults diagnosed with diabetes (138.2 millions) and has also countries with the highest prevalence (Figure (Figure11)[27]. Low- and middle-income countries encompass 80% of the cases, “where the epidemic is gathering pace at alarming rates”[27]. Despite the fact that adult diabetes patients are mainly type 2 patients, it is not clear whether the reported 382 million adults diagnosed with diabetes also include type 1 diabetes patients.
Comparative prevalence of diabetes in adults (20-79 years) in countries with high prevalence (≥ 10%). Data extracted from International Diabetes Federation Diabetes Atlas, 6th ed, 2013.
More than 90%-95% of diabetes patients belong to this type and most of these patients are adults. The number of youth (less than 20 years) with type 2 diabetes in the United States in the year 2009 was 0.46 in 1000 and accounted for approximately 20% of type 2 diabetes in youth[26]. The increased incidence of type 2 diabetes in youth is mainly due to the change in the lifestyle of the children in terms of more sedentary life and less healthy food. Obesity is the major reason behind insulin resistance which is mainly responsible for type 2 diabetes[52-54]. The ADA recommends screening of overweight children and adolescence to detect type 2 diabetes[55,56]. The prevalence of obesity in children in on the rise[6] which is probably the main reason for the increased incidence of type 2 diabetes in the young (30.3% overall increase in type 2 diabetes in children and adolescence between 2001 and 2009)[26].
Insulin resistance in type 2 diabetes patients increases the demand for insulin in insulin-target tissues. In addition to insulin resistance, the increased demand for insulin could not be met by the pancreatic β cells due to defects in the function of these cells[18]. On the contrary, insulin secretion decreases with the increased demand for insulin by time due to the gradual destruction of β cells[57] that could transform some of type 2 diabetes patients from being independent to become dependent on insulin. Most type 2 diabetes patients are not dependent on insulin where insulin secretion continues and insulin depletion rarely occurs. Dependence on insulin is one of the major differences from type 1 diabetes. Other differences include the absence of ketoacidosis in most patients of type 2 diabetes and autoimmune destruction of β cells does not occur. Both type 1 and type 2 diabetes have genetic predisposition, however, it is stronger in type 2 but the genes are more characterized in type 1 (the TCF7L2 gene is strongly associated with type 2 diabetes)[58]. Due to the mild symptoms of type 2 diabetes in the beginning, its diagnosis is usually delayed for years especially in countries where regular checkup without symptoms is not part of the culture. This delay in diagnosis could increase the incidence of long-term complications in type 2 diabetes patients since hyperglycemia is not treated during this undiagnosed period.
In addition to diabetes, insulin resistance has many manifestations that include obesity, nephropathy, essential hypertension, dyslipidemia (hypertriglyceridemia, low HDL, decreased LDL particle diameter, enhanced postprandial lipemia and remnant lipoprotein accumulation), ovarian hyperandrogenism and premature adrenarche, non-alcoholic fatty liver disease and systemic inflammation[6,54]. The presence of type 2 diabetes in children and adolescence who are not obese[59-61], the occasional severe dehydration and the presence of ketoacidosis in some pediatric patients with type 2 diabetes[55] had led to the misclassification of type 2 to type 1 diabetes.
Some patients with many features of type 2 diabetes have some type 1 characteristics including the presence of islet cell autoantibodies or autoantibodies to GAD65 are classified as a distinct type of diabetes called latent autoimmune diabetes in adults (LADA)[62]. People diagnosed with LADA do not require insulin treatment. In a recent study, Hawa et al[63] reported 7.1% of European patients with type 2 diabetes with a mean age of 62 years, tested positive for GAD autoantibodies and the prevalence of LADA was higher in patients diagnosed with diabetes at a younger age. This classification of LADA as a distinct type of diabetes is still controversial[6,64-66].
Insulin resistance and signaling
Defects in the insulin-dependent substrate proteins IRS-1 and IRS-2 mediated signaling pathway are implicated in the development of metabolic disorders, mainly diabetes. This pathway mediates the cellular response to insulin and involves a large array of insulin-stimulated protein kinases including the serine/threonine kinase AKT and protein kinase C (PKC) that phosphorylate a large number of Ser/Thr residues in the insulin receptor substrate (IRS) proteins involved in the metabolic response to insulin[67]. In addition, other non-insulin dependent kinases including the AMP-activated protein kinase, c-Jun N-terminal protein kinase and G protein-coupled receptor kinase 2 that are activated under various conditions can phosphorylate the two insulin responsive substrates[67-71]. Disruption in the AKT and PKC kinases is central to the development of diabetes[72] and is associated with all major features of the disease including hyperinsulinemia, dyslipidemia and insulin resistance[73]. Replacing the wild type IRS-1 with a mutant version of the protein having alanine instead of tyrosine in three locations using genetic knock-in approach provided evidence to the central role of IRS-1 phosphorylation in the development of insulin resistance[74]. Using a similar approach to generate IRS-1 mutant with a single mutation involving a specific tyrosine residue, confirmed the role of IRS-1 phosphorylation in the development of insulin resistance pathogenesis[75]. The large cumulative evidence indicates a complex array of factors including environmental factors[76] and a wide range of cellular disturbances in glucose and lipid metabolism in various tissues[77] contribute to the development of insulin resistance. This condition generates complex cellular metabolic changes in a variety of tissues, mainly liver and muscles, that include the inability of the liver to transport and dispose glucose, control glucose production via gluconeogenesis, impaired storage of glucose as glycogen, de novo lipogenesis and hypertriglyceridemia[77]. Among the factors implicated in the development of insulin resistance, obesity is the most predominant risk factor leading to insulin insensitivity and diabetes which involves several mechanisms that participate in the pathogenesis of the disease[78]. Obesity-induced insulin resistance is directly linked to increased nutrient flux and energy accumulation in tissues that directly affect cell responsiveness to insulin[77]. However, it seems that other insulin-independent mechanisms are involved in the overall metabolic disturbances of glucose homeostasis and diabetes including activities in extra-hepatic tissues in addition to the central role of liver.
OTHER TYPES OF DIABETES MELLITUS
Monogenic diabetes
Characterization of the genetic etiology of diabetes enables more appropriate treatment, better prognosis, and counseling[79]. Monogenic diabetes is due to a genetic defect in single genes in pancreatic β cells which results in disruption of β cell function or a reduction in the number of β cells. Conventionally, monogenic diabetes is classified according to the age of onset as neonatal diabetes before the age of six months or Maturity Onset Diabetes of the Young (MODY) before the age of 25 years. However, certain familial defects are manifested in neonatal diabetes, MODY or adult onset diabetes[2,9,80]. Others believe that classification of diabetes as MODY and neonatal diabetes is obsolete and monogenic diabetes is currently used relating specific genetic etiologies with their specific treatment implications[79]. Beta cell differentiation depends on the expression of the homeodomain transcription factor PDX1 where mutation in the gene results in early onset diabetes (MODY) and its expression decreases before the onset of diabetes[81]. The angiopoietin-like protein 8 (ANGPTL8) may represent a potential “betatrophin” that acts to promote the proliferation of beta cells, however, studies using mice lacking the ANGPTL8 active gene or overexpressed protein indicated that it did not seem to play a role in beta cells proliferation[82].
Mitochondrial diabetes is due to a point mutation in the mitochondrial DNA associated with deafness and maternal transmission of the mutant DNA can result in maternally-inherited diabetes[1,83].
Mutations that result in mutant insulin or the inability to convert proinsulin to insulin result in glucose intolerance in some of these cases. Genetic defects in the insulin receptor or in the signal transduction pathway of insulin have been demonstrated to result in hyperinsulinemia and modest hyperglycemia to severe diabetes[1].
Disease of the exocrine pancreas
Damage of the β cells of the pancreas due to diffused injury of the pancreas can cause diabetes. This damage could be due to pancreatic carcinoma, pancreatitis, infection, pancreatectomy, and trauma[1]. Atrophy of the exocrine pancreas leads to progressive loss of the β cells[84]. Accumulation of fat in the pancreas or pancreatic steatosis could lead to diabetes due to decreased insulin secretion but may require a long time before the damage to β cells occurs[85]. In most cases, extensive damage of the pancreas is required before diabetes occurs and the exocrine function of the pancreas is decreased in these patients[86]. Cirrhosis in cystic fibrosis may contribute to insulin resistance and diabetes[2].
Hormones and drugs
Diabetes has been found in patients with endocrine diseases that secrete excess hormones like growth hormone, glucocorticoids, glucagon and epinephrine in certain endocrinopathies like acromegaly, Cushing’s syndrome, glucagonoma, and pheochromocytoma, respectively[1]. Some of these hormones are used as drugs such as glucocorticoids to suppress the immune system and in chemotherapy and growth hormone to treat children with stunted growth.
Genetic syndromes
Diabetes has been detected in patients with various genetic syndromes such as Down syndrome, Klinefelter syndrome, Turner syndrome and Wolfram syndrome[1].
PREDIABETES
Individuals with prediabetes do not meet the criteria of having diabetes but are at high risk to develop type 2 diabetes in the future. According to the ADA Expert Committee, individuals are defined to have prediabetes if they have either impaired fasting plasma glucose (IFG) levels between 100-125 mg/dL (5.6-6.9 mmol/L) or impaired glucose tolerance test (IGT) with 2-h plasma glucose levels in the oral glucose tolerance test (OGTT) of 140-199 mg/dL (7.8-11.0 mmol/L). The World Health Organization (WHO) still adopts the range for IFG from 110-125 mg/dL (6.1-6.9 mmol/L). Prediabetes has been shown to correlate with increased cardiovascular mortality[87,88] and cancer[89]. The definition of prediabetes with the indicated cut off values is misleading since lower levels of glucose in the normal range are still correlated with cardiovascular disease in a continuous glycemic risk perspective[90]. In accordance with the recommendation of the ADA in 2009 to use hemoglobin A1c (HbA1c) to diagnose diabetes, ADA also recommended the use of an HbA1c (5.7%-6.4%) to diagnose prediabetes[91]. The number of people with IGT according to IDF was 316 million in 2013 (global prevalence 6.9% in adults) and is expected to rise to 471 million in 2030[27]. According to a report in 2014 by the Center for Disease Control and Prevention, 86 million Americans (1 out of 3) have prediabetes[92]. Four of the top ten countries with the highest prevalence of prediabetes are in the Middle East Arab States of the Gulf (Kuwait, Qatar, UAE and Bahrin with prevalence of 17.9%, 17.1%, 16.6% and 16.3%, respectively)[27]. The number of people diagnosed with prediabetes is different according to the method and criteria used to diagnose prediabetes. The number of people with prediabetes defined by IFG 100-125 mg/dL is 4-5 folds higher than those diagnosed using the WHO criteria of 110-125 mg/dL[93]. Diabetes and prediabetes diagnosed using an HbA1c criteria give different estimates compared to methods using FPG or OGTT. Higher percentages of prediabetes were diagnosed using HbA1c compared to FPG[94-96]. Prediabetes is associated with metabolic syndrome and obesity (especially abdominal or visceral obesity), dyslipidemia with high triglycerides and/or low HDL cholesterol, and hypertension[97]. Not all individuals with prediabetes develop diabetes in the future, exercise with a reduction of weight 5%-10% reduces the risk of developing diabetes considerably (40%-70%)[98]. Individuals with an HbA1c of 6.0%-6.5% have twice the risk of developing diabetes (25%-50%) in five years compared to those with an HbA1c of 5.5%-6.0%[99].
DIAGNOSTIC CRITERIA FOR DIABETES MELLITUS
Diabetes mellitus is diagnosed using either the estimation of plasma glucose (FPG or OGTT) or HbA1c. Estimation of the cut off values for glucose and HbA1c is based on the association of FPG or HbA1c with retinopathy. Fasting plasma glucose of ≥ 126 mg/dL (7.0 mmol/L), plasma glucose after 2-h OGTT ≥ 200 mg/dL (11.1 mmol/L), HbA1c ≥ 6.5% (48 mmol/mol) or a random plasma glucose ≥ 200 mg/dL (11.1 mmol/L) along with symptoms of hyperglycemia is diagnostic of diabetes mellitus. In addition to monitor the treatment of diabetes, HbA1c has been recommended to diagnose diabetes by the International Expert Committee in 2009[100] and endorsed by ADA[101], the Endocrine Society, the WHO[102] and many scientists and related organizations all over the world. The advantages and disadvantages of the different tests used to diagnose diabetes have been reviewed by Sacks et al[103]. The advantages of using HbA1c over FPG to diagnose diabetes include greater convenience and preanalytical stability, lower CV (3.6%) compared to FPG (5.7%) and 2h OGTT (16.6%), stronger correlation with microvascular complications especially retinopathy, and a marker for glycemic control and glycation of proteins which is the direct link between diagnosis of diabetes and its complications[104-109]. It is recommended to repeat the HbA1c test in asymptomatic patients within two weeks to reaffirm a single apparently diagnostic result[110].
A cut off value for HbA1c of ≥ 6.5% (48 mmol/mol) has been endorsed by many countries and different ethnic groups, yet ethnicity seems to affect the cut off values to diagnose diabetes[111,112]. Cut-off values of 5.5% (37 mmol/mol)[113] and 6.5% (48 mmol/mol)[114] have been reported in a Japanese study, 6.0% (42 mmol/mol) in the National Health and Nutrition Examination Survey (NHANES III), 6.2% (44 mmol/mol) in a Pima Indian study, 6.3% (45 mmol/mol) in an Egyptian study as reported by Davidson[105]; and three cut-off values for Chinese[112]. The Australians recommended the use of two cut-off values: ≤ 5.5% to “rule-out” and ≥ 7.0% to “rule-in” diabetes[115]. Variations in the prevalence of diabetes[94,116-119] and prediabetes[120] due to ethnicity have been documented. Most studies diagnosed less subjects with diabetes using HbA1c compared to FPG or OGTT[121-123]. Yet, other studies reported more subjects diagnosed with diabetes using HbA1c[96,124-126].
GESTATIONAL DIABETES
Hyperglycemia in pregnancy whether in the form of type 2 diabetes diagnosed before or during pregnancy or in the form gestational diabetes has an increased risk of adverse maternal, fetal and neonatal outcome. Mothers with gestational diabetes and babies born to such mothers have increased risk of developing diabetes later in life. Hyperglycemia in pregnancy is responsible for the increased risk for macrosomia (birth weight ≥ 4.5 kg), large for gestational age births, preeclampsia, preterm birth and cesarean delivery due to large babies[127]. Risk factors for gestational diabetes include obesity, personal history of gestational diabetes, family history of diabetes, maternal age, polycystic ovary syndrome, sedentary life, and exposure to toxic factors[3].
Diagnosis of type 2 diabetes before or during pregnancy is based on criteria mentioned before. Fasting plasma glucose ≥ 126 mg/dL (7.0 mmol/L) or 2-h plasma glucose ≥ 200 mg/dL (11.1 mmol/L) after a 75 g oral glucose load. However, gestational diabetes has been diagnosed at 24-28 wk of gestation in women not previously diagnosed with diabetes using two approaches: the first approach is based on the “one-step” International Association of the Diabetes and Pregnancy Study Groups (IADPSG) consensus[128] and recently adopted by WHO[129]. Gestational diabetes is diagnosed using this method by FPG ≥ 92 mg/dL (5.1 mmol/L), 1-h plasma glucose after a 75 g glucose load ≥ 180 mg/dL (10.0 mmol/L) or 2-h plasma glucose after a 75 g glucose load ≥ 153 mg/dL (8.5 mmol/L). This criteria is derived from the Hyperglycemia and Adverse Pregnancy Outcome (HAPO) study[127] even though the HAPO study showed a continuous relationship between hyperglycemia and adverse short-term pregnancy outcome with no threshold reported[130]. The second approach is used in the United States and is based on the “two-step” NIH consensus[131]. In the first step 1-h plasma glucose after a 50 g glucose load under nonfasting state ≥ 140 mg/dL (7.8 mmol/L) is followed by a second step under fasting conditions after a 100 g glucose load for those who screened abnormal in the first step. The diagnosis of gestational diabetes is made when at least two of the four plasma glucose levels are met. The four plasma glucose levels according to Carpenter/Coustan criteria are: FPG ≥ 95 mg/dL (5.3 mmol/L); 1-h ≥ 180 mg/dL (10.0 mmol/L); 2-h ≥ 155 mg/dL (8.6 mmol/L); and 3-h ≥ 140 mg/dL (7.8 mmol/L)[1].
The use IADPSC criteria in comparison with the Carpenter/Coustan criteria was associated with a 3.5-fold increase in GDM prevalence as well as significant improvements in pregnancy outcomes, and was cost-effective[132]. In another retrospective cohort study of women diagnosed with gestational diabetes, Ethridge et al[133] have shown that newborns of women diagnosed with gestational diabetes by IADPSG approach have greater measures of fetal overgrowth compared with Carpenter-Coustan “two-step” approach neonates. A strategy of using fasting plasma glucose as a screening test and to determine the need for OGTT is valid[134,135]. According to Sacks[136], correlation of glucose concentrations and the risk of subsequent complications will eventually lead to universal guidelines.
The use of ADA/WHO cut off value of HbA1c ≥ 6.5% (48 mmol/mol) to diagnose gestational diabetes is not recommended by the “one step” IADPSC criteria or the “two-step” NIH criteria. Further investigation is required in light of recent reports on HbA1c in combination with OGTT and its usefulness to predict adverse effect of gestational diabetes or obviate the use OGTT in all women with gestational diabetes[137-141].
DIABETES AND GENETICS
Diabetes is a complex disease that involves a wide range of genetic and environmental factors. Over the past several years, many studies have focused on the elucidation of the wide spectrum of genes that played a role in the molecular mechanism of diabetes development[142-144]. However, despite the vast flow of genetic information including the identification of many gene mutations and a large array of single nucleotide polymorphisms (SNPs) in many genes involved in the metabolic pathways that affect blood glucose levels, the exact genetic mechanism of diabetes remains elusive[145,146]. Evidently, a major complication is the fact that a single gene mutation or polymorphism will not impose the same effect among different individuals within a population or different populations. This variation is directly or indirectly affected by the overall genetic background at the individual, family or population levels that are potentially further complicated by interaction with highly variable environmental modifier factors[147,148].
Molecular genetics and type 2 diabetes
One of the major focuses of biomedical research is to delineate the collective and broad genetic variants in the human genome that are involved in the development of diabetes. This major effort will potentially provide the necessary information to understand the molecular genetics of the different forms of diabetes including type 1, type 2 and monogenic neonatal diabetes among individuals of all populations and ethnic groups. Despite the fact that linkage and association studies allowed the identification and characterization of many candidate genes that are associated with type 2 diabetes[144,149,150], however, not all of these genes showed consistent and reproducible association with the disease[151]. Genome wide association studies (GWAS) in various populations identified 70 loci associated with type 2 diabetes and revealed positive linkage of many mutations and SNPs that influence the expression and physiological impact of the related proteins and risk to develop type 2 diabetes. One study involved several thousand type 2 diabetes patients and control subjects from the United Kingdom allowed the identification of several diabetes putative loci positioned in and around the CDKAL1, CDKN2A/B, HHEX/IDE and SLC30A8 genes in addition to the contribution of a large number of other genetic variants that are involved in the development of the disease[152]. Two similar studies from the Finns and Swedish populations and the United States resulted in the identification of similar single nucleotide variants[153] that are linked to the risk of acquiring type 2 diabetes[154,155]. The study in the United States population included in addition to type 2 diabetes, the association of the identified SNPs with the level of triglycerides in the tested subjects[155]. These SNPs are located near several candidate genes including IGFBP2 and CDKAL1 and other genes in addition to several other variants that are located near or in genes firmly associated with the risk of acquiring type 2 diabetes. Other GWAS analysis studies were performed in the Chinese, Malays, and Asian-Indian populations which are distinct from the European and United States populations in addition to meta-analysis of data from other populations in the region revealed relevant findings among patients with European ancestry[156]. The results of the combined analysis showed significant association of SNPs in the CDKAL1, CDKN2A/B, HHEX, KCNQ1 and SLC30A8 genes after adjustment with gender and body mass index. More recently, meta-analysis of GWAS data involving African American type 2 diabetes patients identified similar loci to the previous studies with the addition of two novel loci, HLA-B and INS-IGF[157]. These results provide strong evidence of common genetic determinants including common specific genes that are linked to diabetes. A small list of specific genetic markers seem strongly associated with the risk of developing type 2 diabetes including the TCF7L2[158] and CAPN10[159,160] genes which also play a significant role in the risk and pathogenesis of the disease[158,159]. The association of TCF7L2 gene variants with type 2 diabetes and its mechanism of action received special attention by several investigators[161,162]. Over expression of the protein was shown to decrease the sensitivity of beta islet cells to secrete insulin[163,164] and was more precisely involved in the regulation of secretary granule fusion that constitute a late event in insulin secretion pathway[165]. The role of TCF7L2 in insulin secretion was partially clarified[166] that involves modifying the effect of incretins on insulin secretion by lowering the sensitivity of beta cells to incretins. Several other genes have been found to be significantly associated with the risk of developing type 2 diabetes including a specific SNP in a hematopoietically-expressed homeobox (HHEX) gene[167]. The islet zinc transporter protein (SLC30A8)[168] showed positive correlation with the risk of developing type 2 diabetes where variant mutations in this gene seem protective against the disease which provides a potential tool for therapy[169]. More recently, a low frequency variant of the HNF1A identified by whole exome sequencing was associated with the risk of developing type 2 diabetes among the Latino population and potentially may serve as a screening tool[170]. Genetic variants and specific combined polymorphisms in the interleukin and related genes including interlukin-6 (IL-6), tumor necrosis factor-α and IL-10 genes were found to be associated with greater risk of developing type 2 diabetes[171], in addition to genetic variants in the genes for IL12B, IL23R and IL23A genes[172]. In a study involving the hormone sensitive lipase responsible for lipolysis in adipose tissues, a deletion null mutation, which resulted in the absence of the protein from adipocytes, was reported to be associated with diabetes[173]. Nine specific rare variants in the peroxisome proliferator-activated receptor gamma (PPARG) gene that resulted in loss of the function of the protein in adipocytes differentiation, were significantly associated with the risk of developing type 2 diabetes[174]. In addition, certain SNPs in the alpha 2A adrenergic receptor (ADRA2A) gene, involved in the sympathetic nervous system control of insulin secretion and lipolysis, were found to be associated with obesity and type 2 diabetes[175]. Link analysis between the melatonin MT2 receptor (MTNR1B) gene, a G-protein coupled receptor, identified 14 mutant variants from 40 known variants revealed by exome sequencing, to be positively linked with type 2 diabetes[176]. The authors suggested that mutations in the MT2 gene could provide a tool with other related genes in modifying therapy for type 2 diabetes patients based on their specific genetic background to formulate personalized therapies which potentially may ensures the optimum response. Interestingly, mutations in the clock[177,178] and Bmal1[179] transcription factor genes which are involved in beta cells biological clock affecting growth, survival and synaptic vesicle assembly in these cells, resulted in reduced insulin secretion and diabetes. Evidently, prominent metabolic functions involve the production of specific reactive metabolites, leading to oxidative stress, which affect lipids, proteins and other biological compounds leading to serious damage in various tissues and organs. Mutations and SNPs in the antioxidant genes, including superoxide dismutase, catalase and glutathione peroxidase, that decrease their activity are implicated in the risk and pathogenesis of type 2 diabetes[180]. The metabolic syndrome was shown to be associated with the development of type 2 diabetes in a population that is described as highly endogenous especially in individuals over 45 years of age[181]. Since consanguinity marriages is high in this population, screening for this syndrome among families could provide an informative marker on the risk of developing type 2 diabetes[181].
Molecular genetics of type 1 diabetes
Even though type 1 diabetes is basically described as an autoimmune disease that results in the destruction of pancreatic beta cells, however, single gene mutations and SNPs have been found to be associated with the susceptibility to this type of diabetes. Initially, two gene mutations were linked to the development of type 1 diabetes including the autoimmune regulator (AIRE) gene which affect the immune tolerance to self antigens leading to autoimmunity[182] and the FOXP3 gene which results in defective regulatory T cells[183]. In addition, a mutation in the histone deacetylase SIRTI gene predominantly expressed in beta cells involved in the regulation of insulin secretion[184] and played a role in modulating the sensitivity of peripheral tissues to insulin[185] was detected in type 1 diabetes patients[186]. Recently, additional mutations and SNPs in the CTLA-4 +49A/G and HLA-DQB1 and INS gene VNTR alleles were found to be associated with type 1 diabetes, which have the advantage of differentiating between Latent autoimmune type 1 diabetes and type 2 diabetes[187]. The HLA-DQB1, in combination with HLA-DR alleles and a polymorphism in PTPN22 gene seem to be associated with the age onset of late type 1 diabetes[188,189]. Two specific polymorphisms in the promoter region of a transmembrane protein (DC-SIGN) gene expressed in macrophages and played an important role of T- cell activation and inflammation were found to be protective against type 1 diabetes[190]. An innovative non-parametric SNP enrichment tool using summary GWAS DATA allowed the identification of association between several transcription factors and type 1 diabetes and are located in a type 1 diabetes susceptibility region[191]. Nine SNP variants in several genes associated with type 1 diabetes, not including the major histocompatibility gene region, were identified using extensive GWAS analysis[192]. Furthermore, several novel SNPs in a region in chromosome 16 located in the CLEC16A gene were shown to be associated with type 1 diabetes and seem to function through the reduced expression of DEX1 in B lymphoblastoid cells[193]. Since more than 40 regions in the human genome were identified to be associated with the susceptibility to type 1 diabetes[194-196], a weighted risk model was developed utilizing selected genes SNPs could be used for testing infants for these genetic markers that could provide insights in the susceptibility to type 1 diabetes development or safe prevention of the disease among young children[197].
Molecular genetics of monogenic diabetes
A large array of genes were identified to be involved in the development of monogenic diabetes[80] which represent about 2%-5% of diabetes patients. Monogenic diabetes results primarily from gene defects that lead to a decrease in beta cell number or function. Monogenic diabetes genes were identified using linkage studies or code for proteins that directly affected glucose homeostasis. The majority of genes responsible for monogenetic diabetes code for either transcription factors that participate in the control of nuclear gene expression or proteins that are located on the cell membrane, cytoplasm and endoplasmic reticulum, proteins involved in insulin synthesis and secretion, exocrine pancreatic proteins and autoimmune diabetes proteins[80]. The collective function of these proteins is their participation in glucose metabolism at different levels. Evidently, the hierarchy of a specific gene in the overall glucose metabolism pathway determines the onset of diabetes in the patient and whether it is neonataly expressed or have late onset expression (adulthood). Consequently, molecular defects in the structure and function of these genes lead to the disturbance of plasma glucose level, the primary pathological sign of diabetes. The molecular mechanism of permanent neonatal diabetes mellitus (PNDP) in addition to MODY explains the observed phenotype of monogenetic diabetes that involves loss of function of the expressed mutant protein. The first gene implicated in monogenic diabetes was the glucokinase (GCK) gene[198] which functions as a pancreatic sensor for blood glucose where more than 70 mutations in the gene were identified that affected its activity[199]. A recent study on GCK gene mutations causing neonatal and childhood diabetes showed that the majority of mutations resulted in the loss of the enzyme function primarily due to protein instability[148,150]. Two hepatocytes nuclear factor genes that code for the HNF4A and HNF1A transcription factors were closely associated with MODY1 and MODY2[148,149]. Definitely, a whole list of other genes involved in monogenic diabetes are either overlooked or included in the genetic determinants of type 1 and type 2 diabetes which will be identified and clarified through more careful future studies.
MOLECULAR GENETICS OF DIABETES COMPLICATIONS
In addition to the genetic determinants of diabetes, several gene mutations and polymorphisms have been associated with the clinical complications of diabetes. The cumulative data on diabetes patients with a variety of micro- and macrovascular complications support the presence of strong genetic factors involved in the development of various complications[200]. A list of genes have been reported that are associated with diabetes complications including ACE and AKR1B1 in nephropathy, VEGF and AKRB1 in retinopathy and ADIPOQ and GLUL in cardiovascular diseases[200]. A study on Chinese patients revealed a single SNP in the promoter region of the smooth muscle actin (ACTA2) gene correlates with the degree of coronary artery stenosis in type 2 diabetes patients[201]. Furthermore, the alpha kinase 1 gene (ALPK1) identified as a susceptibility gene for chronic kidney disease by GWAS[202], was demonstrated in type 2 diabetes patients[203]. Three additional genes have been strongly correlated with this risk of diabetic retinopathy (DR) including the vascular endothelial growth receptor, aldose reductase and the receptor for advanced glycation products genes[204] where specific polymorphisms in these genes seem to increase the risk of DR development in diabetes patients[204]. A significant differential proteome (involving 56 out of 252 proteins) is evident that characterizes vitreous samples obtained from diabetes patients with the complication in comparison to diabetes patients without the complication and control individuals[205]. Interestingly, a large portion of these proteins (30 proteins) belong to the kallikrein-kinin, coagulation and complement systems including complement C3, complement factor 1, prothrombin, alpha-1-antitrypsin and antithrombin III that are elevated in diabetic patients with retinopathy[205]. In addition, 2 single nucleotides polymorphisms in the human related B7-I gene seem to mediate podocyte injury in diabetic nephropathy[206]. Furthermore, increased concentration of the ligand of B7-1 correlates with the progression of end-stage renal disease (ESRD) in diabetes patients[206]. These results indicate that B7-I inhibition may serve as a potential target for diabetes nephropathy prevention and/or treatment. Recently, it was shown that direct correlation is evident between circulating levels of tumor necrosis factors 1 and 2 and increased risk of ESRD in American Indian patients[207]. The link between diabetes and proper bone development and health is evident. Studies using animal models with major significant reduction in insulin receptor (IR) in osteoprogenitor cells resulted in thin and rod-like weak bones with high risk of fractures[208]. Similar findings were observed in animal models with bone-specific IR knockdown animals which points to the central role of IR in the proper development of bones[208]. Type 2 diabetes is also associated with mitochondrial dysfunction in adipose tissues. Using knockout animal models of specific mitochondrial genes led to significant reduction in key electron transport complexes expression and eventually adipocytes death[209]. These animals exhibited Insulin resistance in addition to other complications that can potentially lead to cardiovascular disease[209].
CONCLUSION
Diabetes mellitus is the epidemic of the century and without effective diagnostic methods at an early stage, diabetes will continue to rise. This review focuses on the types of diabetes and the effective diagnostic methods and criteria to be used for diagnosis of diabetes and prediabetes. Evidently, diabetes is a complex disease with a large pool of genes that are involved in its development. The precise identification of the genetic bases of diabetes potentially provides an essential tool to improve diagnoses, therapy (more towards individualized patient targeted therapy) and better effective genetic counseling. Furthermore, our advanced knowledge of the association between medical genetics and the chronic complications of diabetes, will provide an additional advantage to delay or eradicate these complications that impose an immense pressure on patient’s quality of life and the significantly rising cost of health-care services.
Footnotes
Conflict-of-interest: The authors declare that there is no conflict of interest associated with this manuscript.
Open-Access: This article is an open-access article which was selected by an in-house editor and fully peer-reviewed by external reviewers. It is distributed in accordance with the Creative Commons Attribution Non Commercial (CC BY-NC 4.0) license, which permits others to distribute, remix, adapt, build upon this work non-commercially, and license their derivative works on different terms, provided the original work is properly cited and the use is non-commercial. See: http://creativecommons.org/licenses/by-nc/4.0/
Peer-review started: November 23, 2014
First decision: February 7, 2015
Article in press: April 14, 2015
P- Reviewer: Hegardt FG, Surani S, Traub M S- Editor: Gong XM L- Editor: A E- Editor: Wang CH
Article information
World J Diabetes. 2015 Jun 25; 6(6): 850–867.
Published online 2015 Jun 25. doi: 10.4239/wjd.v6.i6.850
PMCID: PMC4478580
PMID: 26131326
Akram T Kharroubi and Hisham M Darwish
Akram T Kharroubi, Department of Medical Laboratory Sciences, Faculty of Health Professions, Al-Quds University, Jerusalem 91000, Palestine
Hisham M Darwish, Department of Biochemistry, Faculty of Medicine, Al-Quds University, Jerusalem 91000, Palestine
Author contributions: Kharroubi AT and Darwish HM contributed equally to the writing of the review article; Kharroubi AT wrote the classification, diagnosis, and etiology of diabetes; Darwish HM wrote the molecular genetics of diabetes.
Correspondence to: Akram T Kharroubi, PhD, Associate Professor of Biochemistry and Endocrinology, Dean of Faculty of Health Professions, Department of Medical Laboratory Sciences, Faculty of Health Professions, Al-Quds University, P.O. Box 51000, Abed Elhamaid Shoman Street, Beit Hanina-Jerusalem, Jerusalem 91000, Palestine. moc.liamg@ibuorrahk.marka
Telephone: +972-2-2791243 Fax: +972-2-2791243
Received 2014 Nov 20; Revised 2015 Mar 25; Accepted 2015 Apr 10.
Copyright ©The Author(s) 2015. Published by Baishideng Publishing Group Inc. All rights reserved.
This article has been cited by other articles in PMC.
Articles from World Journal of Diabetes are provided here courtesy of Baishideng Publishing Group Inc
References
1. American Diabetes Association. Diagnosis and classification of diabetes mellitus. Diabetes Care. 2014;37 Suppl 1:S81–S90. [PubMed] [Google Scholar]
2. Craig ME, Hattersley A, Donaghue KC. Definition, epidemiology and classification of diabetes in children and adolescents. Pediatr Diabetes. 2009;10 Suppl 12:3–12. [PubMed] [Google Scholar]
3. Galtier F. Definition, epidemiology, risk factors. Diabetes Metab. 2010;36:628–651. [PubMed] [Google Scholar]
4. Thunander M, Törn C, Petersson C, Ossiansson B, Fornander J, Landin-Olsson M. Levels of C-peptide, body mass index and age, and their usefulness in classification of diabetes in relation to autoimmunity, in adults with newly diagnosed diabetes in Kronoberg, Sweden. Eur J Endocrinol. 2012;166:1021–1029. [PMC free article] [PubMed] [Google Scholar]
5. Stone MA, Camosso-Stefinovic J, Wilkinson J, de Lusignan S, Hattersley AT, Khunti K. Incorrect and incomplete coding and classification of diabetes: a systematic review. Diabet Med. 2010;27:491–497. [PubMed] [Google Scholar]
6. Rosenbloom AL, Silverstein JH, Amemiya S, Zeitler P, Klingensmith GJ. Type 2 diabetes in children and adolescents. Pediatr Diabetes. 2009;10 Suppl 12:17–32. [PubMed] [Google Scholar]
7. Cakan N, Kizilbash S, Kamat D. Changing spectrum of diabetes mellitus in children: challenges with initial classification. Clin Pediatr (Phila) 2012;51:939–944. [PubMed] [Google Scholar]
8. Wilkin TJ. The accelerator hypothesis: a review of the evidence for insulin resistance as the basis for type I as well as type II diabetes. Int J Obes (Lond) 2009;33:716–726. [PubMed] [Google Scholar]
9. Canivell S, Gomis R. Diagnosis and classification of autoimmune diabetes mellitus. Autoimmun Rev. 2014;13:403–407. [PubMed] [Google Scholar]
10. Lamb MM, Yin X, Zerbe GO, Klingensmith GJ, Dabelea D, Fingerlin TE, Rewers M, Norris JM. Height growth velocity, islet autoimmunity and type 1 diabetes development: the Diabetes Autoimmunity Study in the Young. Diabetologia. 2009;52:2064–2071. [PMC free article] [PubMed] [Google Scholar]
11. Vehik K, Hamman RF, Lezotte D, Norris JM, Klingensmith GJ, Dabelea D. Childhood growth and age at diagnosis with Type 1 diabetes in Colorado young people. Diabet Med. 2009;26:961–967. [PubMed] [Google Scholar]
12. Ferrannini E, Mari A, Nofrate V, Sosenko JM, Skyler JS; DPT-1 Study Group. Progression to diabetes in relatives of type 1 diabetic patients: mechanisms and mode of onset. Diabetes. 2010;59:679–685. [PMC free article] [PubMed] [Google Scholar]
13. Robertson RP, Harmon J, Tran PO, Tanaka Y, Takahashi H. Glucose toxicity in beta-cells: type 2 diabetes, good radicals gone bad, and the glutathione connection. Diabetes. 2003;52:581–587. [PubMed] [Google Scholar]
14. Vincent AM, Russell JW, Low P, Feldman EL. Oxidative stress in the pathogenesis of diabetic neuropathy. Endocr Rev. 2004;25:612–628. [PubMed] [Google Scholar]
15. Giugliano D, Ceriello A, Paolisso G. Oxidative stress and diabetic vascular complications. Diabetes Care. 1996;19:257–267. [PubMed] [Google Scholar]
16. Giacco F, Brownlee M. Oxidative stress and diabetic complications. Circ Res. 2010;107:1058–1070. [PMC free article] [PubMed] [Google Scholar]
17. Elmarakby AA, Sullivan JC. Relationship between oxidative stress and inflammatory cytokines in diabetic nephropathy. Cardiovasc Ther. 2012;30:49–59. [PubMed] [Google Scholar]
18. Halban PA, Polonsky KS, Bowden DW, Hawkins MA, Ling C, Mather KJ, Powers AC, Rhodes CJ, Sussel L, Weir GC. β-cell failure in type 2 diabetes: postulated mechanisms and prospects for prevention and treatment. Diabetes Care. 2014;37:1751–1758. [PMC free article] [PubMed] [Google Scholar]
19. Johansen JS, Harris AK, Rychly DJ, Ergul A. Oxidative stress and the use of antioxidants in diabetes: linking basic science to clinical practice. Cardiovasc Diabetol. 2005;4:5. [PMC free article] [PubMed] [Google Scholar]
20. Kaneto H, Kajimoto Y, Miyagawa J, Matsuoka T, Fujitani Y, Umayahara Y, Hanafusa T, Matsuzawa Y, Yamasaki Y, Hori M. Beneficial effects of antioxidants in diabetes: possible protection of pancreatic beta-cells against glucose toxicity. Diabetes. 1999;48:2398–2406. [PubMed] [Google Scholar]
21. Nebbioso M, Federici M, Rusciano D, Evangelista M, Pescosolido N. Oxidative stress in preretinopathic diabetes subjects and antioxidants. Diabetes Technol Ther. 2012;14:257–263. [PubMed] [Google Scholar]
22. Kilic G, Alvarez-Mercado AI, Zarrouki B, Opland D, Liew CW, Alonso LC, Myers MG, Jonas JC, Poitout V, Kulkarni RN, et al. The islet estrogen receptor-α is induced by hyperglycemia and protects against oxidative stress-induced insulin-deficient diabetes. PLoS One. 2014;9:e87941. [PMC free article] [PubMed] [Google Scholar]
23. Maahs DM, West NA, Lawrence JM, Mayer-Davis EJ. Epidemiology of type 1 diabetes. Endocrinol Metab Clin North Am. 2010;39:481–497. [PMC free article] [PubMed] [Google Scholar]
24. Daneman D. Type 1 diabetes. Lancet. 2006;367:847–858. [PubMed] [Google Scholar]
25. Devendra D, Liu E, Eisenbarth GS. Type 1 diabetes: recent developments. BMJ. 2004;328:750–754. [PMC free article] [PubMed] [Google Scholar]
26. Dabelea D, Mayer-Davis EJ, Saydah S, Imperatore G, Linder B, Divers J, Bell R, Badaru A, Talton JW, Crume T, et al. Prevalence of type 1 and type 2 diabetes among children and adolescents from 2001 to 2009. JAMA. 2014;311:1778–1786. [PMC free article] [PubMed] [Google Scholar]
27. International Diabetes Federation. IDF Diabetes Atlas. 6th ed. Brussels, Belgium: International Diabetes Federation; 2013. [Google Scholar]
28. Prime Group for JDRF. JDRF: Type 1 Diabetes; 2011. [Google Scholar]
29. Chiang JL, Kirkman MS, Laffel LM, Peters AL; Type 1 Diabetes Sourcebook Authors. Type 1 diabetes through the life span: a position statement of the American Diabetes Association. Diabetes Care. 2014;37:2034–2054. [PMC free article] [PubMed] [Google Scholar]
30. Pettitt DJ, Talton J, Dabelea D, Divers J, Imperatore G, Lawrence JM, Liese AD, Linder B, Mayer-Davis EJ, Pihoker C, et al. Prevalence of diabetes in U.S. youth in 2009: the SEARCH for diabetes in youth study. Diabetes Care. 2014;37:402–408. [PMC free article] [PubMed] [Google Scholar]
31. Lawrence JM, Imperatore G, Dabelea D, Mayer-Davis EJ, Linder B, Saydah S, Klingensmith GJ, Dolan L, Standiford DA, Pihoker C, et al. Trends in incidence of type 1 diabetes among non-Hispanic white youth in the U.S., 2002-2009. Diabetes. 2014;63:3938–3945. [PMC free article] [PubMed] [Google Scholar]
32. Vermeulen I, Weets I, Asanghanwa M, Ruige J, Van Gaal L, Mathieu C, Keymeulen B, Lampasona V, Wenzlau JM, Hutton JC, et al. Contribution of antibodies against IA-2β and zinc transporter 8 to classification of diabetes diagnosed under 40 years of age. Diabetes Care. 2011;34:1760–1765. [PMC free article] [PubMed] [Google Scholar]
33. Couper J, Donaghue KC. Phases of diabetes in children and adolescents. Pediatr Diabetes. 2009;10 Suppl 12:13–16. [PubMed] [Google Scholar]
34. Ginsberg-Fellner F, Witt ME, Fedun B, Taub F, Dobersen MJ, McEvoy RC, Cooper LZ, Notkins AL, Rubinstein P. Diabetes mellitus and autoimmunity in patients with the congenital rubella syndrome. Rev Infect Dis. 1985;7 Suppl 1:S170–S176. [PubMed] [Google Scholar]
35. McIntosh ED, Menser MA. A fifty-year follow-up of congenital rubella. Lancet. 1992;340:414–415. [PubMed] [Google Scholar]
36. Stene LC, Oikarinen S, Hyöty H, Barriga KJ, Norris JM, Klingensmith G, Hutton JC, Erlich HA, Eisenbarth GS, Rewers M. Enterovirus infection and progression from islet autoimmunity to type 1 diabetes: the Diabetes and Autoimmunity Study in the Young (DAISY) Diabetes. 2010;59:3174–3180. [PMC free article] [PubMed] [Google Scholar]
37. Yeung WC, Rawlinson WD, Craig ME. Enterovirus infection and type 1 diabetes mellitus: systematic review and meta-analysis of observational molecular studies. BMJ. 2011;342:d35. [PMC free article] [PubMed] [Google Scholar]
38. Hyppönen E, Läärä E, Reunanen A, Järvelin MR, Virtanen SM. Intake of vitamin D and risk of type 1 diabetes: a birth-cohort study. Lancet. 2001;358:1500–1503. [PubMed] [Google Scholar]
39. Knip M, Virtanen SM, Seppä K, Ilonen J, Savilahti E, Vaarala O, Reunanen A, Teramo K, Hämäläinen AM, Paronen J, et al. Dietary intervention in infancy and later signs of beta-cell autoimmunity. N Engl J Med. 2010;363:1900–1908. [PMC free article] [PubMed] [Google Scholar]
40. Forlenza GP, Rewers M. The epidemic of type 1 diabetes: what is it telling us? Curr Opin Endocrinol Diabetes Obes. 2011;18:248–251. [PubMed] [Google Scholar]
41. Ferreira RC, Guo H, Coulson RM, Smyth DJ, Pekalski ML, Burren OS, Cutler AJ, Doecke JD, Flint S, McKinney EF, et al. A type I interferon transcriptional signature precedes autoimmunity in children genetically at risk for type 1 diabetes. Diabetes. 2014;63:2538–2550. [PMC free article] [PubMed] [Google Scholar]
42. Kallionpää H, Elo LL, Laajala E, Mykkänen J, Ricaño-Ponce I, Vaarma M, Laajala TD, Hyöty H, Ilonen J, Veijola R, et al. Innate immune activity is detected prior to seroconversion in children with HLA-conferred type 1 diabetes susceptibility. Diabetes. 2014;63:2402–2414. [PubMed] [Google Scholar]
43. Richardson SJ, Horwitz MS. Is type 1 diabetes “going viral”? Diabetes. 2014;63:2203–2205. [PubMed] [Google Scholar]
44. Lombardo F, Valenzise M, Wasniewska M, Messina MF, Ruggeri C, Arrigo T, De Luca F. Two-year prospective evaluation of the factors affecting honeymoon frequency and duration in children with insulin dependent diabetes mellitus: the key-role of age at diagnosis. Diabetes Nutr Metab. 2002;15:246–251. [PubMed] [Google Scholar]
45. Abiru N, Kawasaki E, Eguch K. Current knowledge of Japanese type 1 diabetic syndrome. Diabetes Metab Res Rev. 2002;18:357–366. [PubMed] [Google Scholar]
46. Imagawa A, Hanafusa T, Miyagawa J, Matsuzawa Y. A proposal of three distinct subtypes of type 1 diabetes mellitus based on clinical and pathological evidence. Ann Med. 2000;32:539–543. [PubMed] [Google Scholar]
47. Imagawa A, Hanafusa T, Miyagawa J, Matsuzawa Y. A novel subtype of type 1 diabetes mellitus characterized by a rapid onset and an absence of diabetes-related antibodies. Osaka IDDM Study Group. N Engl J Med. 2000;342:301–307. [PubMed] [Google Scholar]
48. Imagawa A, Hanafusa T. Fulminant type 1 diabetes--an important subtype in East Asia. Diabetes Metab Res Rev. 2011;27:959–964. [PubMed] [Google Scholar]
49. Shibasaki S, Imagawa A, Hanafusa T. Fulminant type 1 diabetes mellitus: a new class of type 1 diabetes. Adv Exp Med Biol. 2012;771:20–23. [PubMed] [Google Scholar]
50. Imagawa A, Hanafusa T. Fulminant type 1 diabetes mellitus. Endocr J. 2006;53:577–584. [PubMed] [Google Scholar]
51. Shimizu I, Makino H, Imagawa A, Iwahashi H, Uchigata Y, Kanatsuka A, Kawasaki E, Kobayashi T, Shimada A, Maruyama T, et al. Clinical and immunogenetic characteristics of fulminant type 1 diabetes associated with pregnancy. J Clin Endocrinol Metab. 2006;91:471–476. [PubMed] [Google Scholar]
52. Ginsberg H, Kimmerling G, Olefsky JM, Reaven GM. Demonstration of insulin resistance in untreated adult onset diabetic subjects with fasting hyperglycemia. J Clin Invest. 1975;55:454–461. [PMC free article] [PubMed] [Google Scholar]
53. Olefsky J, Farquhar JW, Reaven G. Relationship between fasting plasma insulin level and resistance to insulin-mediated glucose uptake in normal and diabetic subjects. Diabetes. 1973;22:507–513. [PubMed] [Google Scholar]
54. Kraemer FB, Ginsberg HN. Gerald M. Reaven, MD: Demonstration of the central role of insulin resistance in type 2 diabetes and cardiovascular disease. Diabetes Care. 2014;37:1178–1181. [PubMed] [Google Scholar]
55. Type 2 diabetes in children and adolescents. American Diabetes Association. Diabetes Care. 2000;23:381–389. [PubMed] [Google Scholar]
56. Reinehr T. Type 2 diabetes mellitus in children and adolescents. World J Diabetes. 2013;4:270–281. [PMC free article] [PubMed] [Google Scholar]
57. Druet C, Tubiana-Rufi N, Chevenne D, Rigal O, Polak M, Levy-Marchal C. Characterization of insulin secretion and resistance in type 2 diabetes of adolescents. J Clin Endocrinol Metab. 2006;91:401–404. [PubMed] [Google Scholar]
58. Saadi H, Nagelkerke N, Carruthers SG, Benedict S, Abdulkhalek S, Reed R, Lukic M, Nicholls MG. Association of TCF7L2 polymorphism with diabetes mellitus, metabolic syndrome, and markers of beta cell function and insulin resistance in a population-based sample of Emirati subjects. Diabetes Res Clin Pract. 2008;80:392–398. [PubMed] [Google Scholar]
59. Wei JN, Sung FC, Li CY, Chang CH, Lin RS, Lin CC, Chiang CC, Chuang LM. Low birth weight and high birth weight infants are both at an increased risk to have type 2 diabetes among schoolchildren in taiwan. Diabetes Care. 2003;26:343–348. [PubMed] [Google Scholar]
60. Ramachandran A, Snehalatha C, Satyavani K, Sivasankari S, Vijay V. Type 2 diabetes in Asian-Indian urban children. Diabetes Care. 2003;26:1022–1025. [PubMed] [Google Scholar]
61. Sugihara S, Sasaki N, Kohno H, Amemiya S, Tanaka T, Matsuura N; Committee for the Medical Treatment of Childhood-Onset Type 2 Diabetes Mellitus, The Japanese Society for Pediatric Endocrinology. Survey of current medical treatments for childhood-onset type 2 diabetes mellitus in Japan. Clin Pediatr Endocrinol. 2005;14:65–75. [PMC free article] [PubMed] [Google Scholar]
62. Pozzilli P, Di Mario U. Autoimmune diabetes not requiring insulin at diagnosis (latent autoimmune diabetes of the adult): definition, characterization, and potential prevention. Diabetes Care. 2001;24:1460–1467. [PubMed] [Google Scholar]
63. Hawa MI, Buchan AP, Ola T, Wun CC, DeMicco DA, Bao W, Betteridge DJ, Durrington PN, Fuller JH, Neil HA, et al. LADA and CARDS: a prospective study of clinical outcome in established adult-onset autoimmune diabetes. Diabetes Care. 2014;37:1643–1649. [PubMed] [Google Scholar]
64. Rolandsson O, Palmer JP. Latent autoimmune diabetes in adults (LADA) is dead: long live autoimmune diabetes! Diabetologia. 2010;53:1250–1253. [PubMed] [Google Scholar]
65. Redondo MJ. LADA: time for a new definition. Diabetes. 2013;62:339–340. [PMC free article] [PubMed] [Google Scholar]
66. Leslie RD, Kolb H, Schloot NC, Buzzetti R, Mauricio D, De Leiva A, Yderstraede K, Sarti C, Thivolet C, Hadden D, et al. Diabetes classification: grey zones, sound and smoke: Action LADA 1. Diabetes Metab Res Rev. 2008;24:511–519. [PubMed] [Google Scholar]
67. Copps KD, White MF. Regulation of insulin sensitivity by serine/threonine phosphorylation of insulin receptor substrate proteins IRS1 and IRS2. Diabetologia. 2012;55:2565–2582. [PMC free article] [PubMed] [Google Scholar]
68. Boura-Halfon S, Zick Y. Phosphorylation of IRS proteins, insulin action, and insulin resistance. Am J Physiol Endocrinol Metab. 2009;296:E581–E591. [PubMed] [Google Scholar]
69. Tanti JF, Jager J. Cellular mechanisms of insulin resistance: role of stress-regulated serine kinases and insulin receptor substrates (IRS) serine phosphorylation. Curr Opin Pharmacol. 2009;9:753–762. [PubMed] [Google Scholar]
70. Cheng Z, Tseng Y, White MF. Insulin signaling meets mitochondria in metabolism. Trends Endocrinol Metab. 2010;21:589–598. [PMC free article] [PubMed] [Google Scholar]
71. White MF. Insulin signaling in health and disease. Science. 2003;302:1710–1711. [PubMed] [Google Scholar]
72. Farese RV, Sajan MP, Standaert ML. Insulin-sensitive protein kinases (atypical protein kinase C and protein kinase B/Akt): actions and defects in obesity and type II diabetes. Exp Biol Med (Maywood) 2005;230:593–605. [PubMed] [Google Scholar]
73. Farese RV, Sajan MP, Yang H, Li P, Mastorides S, Gower WR, Nimal S, Choi CS, Kim S, Shulman GI, et al. Muscle-specific knockout of PKC-lambda impairs glucose transport and induces metabolic and diabetic syndromes. J Clin Invest. 2007;117:2289–2301. [PMC free article] [PubMed] [Google Scholar]
74. Morino K, Neschen S, Bilz S, Sono S, Tsirigotis D, Reznick RM, Moore I, Nagai Y, Samuel V, Sebastian D, et al. Muscle-specific IRS-1 Ser-& gt; Ala transgenic mice are protected from fat-induced insulin resistance in skeletal muscle. Diabetes. 2008;57:2644–2651. [PMC free article] [PubMed] [Google Scholar]
75. Copps KD, Hancer NJ, Opare-Ado L, Qiu W, Walsh C, White MF. Irs1 serine 307 promotes insulin sensitivity in mice. Cell Metab. 2010;11:84–92. [PMC free article] [PubMed] [Google Scholar]
76. Sargis RM. The hijacking of cellular signaling and the diabetes epidemic: mechanisms of environmental disruption of insulin action and glucose homeostasis. Diabetes Metab J. 2014;38:13–24. [PMC free article] [PubMed] [Google Scholar]
77. Otero YF, Stafford JM, McGuinness OP. Pathway-selective insulin resistance and metabolic disease: the importance of nutrient flux. J Biol Chem. 2014;289:20462–20469. [PMC free article] [PubMed] [Google Scholar]
78. Ye J. Mechanisms of insulin resistance in obesity. Front Med. 2013;7:14–24. [PMC free article] [PubMed] [Google Scholar]
79. Murphy R, Ellard S, Hattersley AT. Clinical implications of a molecular genetic classification of monogenic beta-cell diabetes. Nat Clin Pract Endocrinol Metab. 2008;4:200–213. [PubMed] [Google Scholar]
80. Schwitzgebel VM. Many faces of monogenic diabetes. J Diabetes Investig. 2014;5:121–133. [PMC free article] [PubMed] [Google Scholar]
81. Kushner JA, Ye J, Schubert M, Burks DJ, Dow MA, Flint CL, Dutta S, Wright CV, Montminy MR, White MF. Pdx1 restores beta cell function in Irs2 knockout mice. J Clin Invest. 2002;109:1193–1201. [PMC free article] [PubMed] [Google Scholar]
82. Gusarova V, Alexa CA, Na E, Stevis PE, Xin Y, Bonner-Weir S, Cohen JC, Hobbs HH, Murphy AJ, Yancopoulos GD, et al. ANGPTL8/betatrophin does not control pancreatic beta cell expansion. Cell. 2014;159:691–696. [PMC free article] [PubMed] [Google Scholar]
83. Reardon W, Ross RJ, Sweeney MG, Luxon LM, Pembrey ME, Harding AE, Trembath RC. Diabetes mellitus associated with a pathogenic point mutation in mitochondrial DNA. Lancet. 1992;340:1376–1379. [PubMed] [Google Scholar]
84. Chen N, Unnikrishnan I R, Anjana RM, Mohan V, Pitchumoni CS. The complex exocrine-endocrine relationship and secondary diabetes in exocrine pancreatic disorders. J Clin Gastroenterol. 2011;45:850–861. [PubMed] [Google Scholar]
85. Pezzilli R, Calculli L. Pancreatic steatosis: Is it related to either obesity or diabetes mellitus? World J Diabetes. 2014;5:415–419. [PMC free article] [PubMed] [Google Scholar]
86. Larger E, Philippe MF, Barbot-Trystram L, Radu A, Rotariu M, Nobécourt E, Boitard C. Pancreatic exocrine function in patients with diabetes. Diabet Med. 2012;29:1047–1054. [PubMed] [Google Scholar]
87. Huang Y, Cai X, Chen P, Mai W, Tang H, Huang Y, Hu Y. Associations of prediabetes with all-cause and cardiovascular mortality: a meta-analysis. Ann Med. 2014;46:684–692. [PubMed] [Google Scholar]
88. Sherwin R, Jastreboff AM. Year in diabetes 2012: The diabetes tsunami. J Clin Endocrinol Metab. 2012;97:4293–4301. [PMC free article] [PubMed] [Google Scholar]
89. Huang Y, Cai X, Qiu M, Chen P, Tang H, Hu Y, Huang Y. Prediabetes and the risk of cancer: a meta-analysis. Diabetologia. 2014;57:2261–2269. [PubMed] [Google Scholar]
90. Buysschaert M, Bergman M. Definition of prediabetes. Med Clin North Am. 2011;95:289–297, vii. [PubMed] [Google Scholar]
91. American Diabetes Association. Diagnosis and classification of diabetes mellitus. Diabetes Care. 2010;33 Suppl 1:S62–S69. [PMC free article] [PubMed] [Google Scholar]
92. Center for Disease Control and Prevention. National diabetes statistics report: Estimates of diabetes and its burden in the United States. Atlanta, GA: US Department of Health and Human Services; 2014. [Google Scholar]
93. Inoue K, Matsumoto M, Akimoto K. The threshold for definition of impaired fasting glucose in a Japanese population. Diabet Med. 2009;26:1175–1178. [PubMed] [Google Scholar]
94. Bernal-Lopez MR, Santamaría-Fernandez S, Lopez-Carmona D, Tinahones FJ, Mancera-Romero J, Peña-Jimenez D, Jansen-Chaparro S, Baca-Osorio AJ, Cuesta-Muñoz AL, Serrano-Rios M, et al. HbA(1c) in adults without known diabetes from southern Europe. Impact of the new diagnostic criteria in clinical practice. Diabet Med. 2011;28:1319–1322. [PubMed] [Google Scholar]
95. Bersoux S, Cook CB, Wu Q, Burritt MF, Hernandez JS, Verona PM, Larson MH, LaRosa CS. Hemoglobin A1c testing alone does not sufficiently identify patients with prediabetes. Am J Clin Pathol. 2011;135:674–677. [PubMed] [Google Scholar]
96. Lipska KJ, De Rekeneire N, Van Ness PH, Johnson KC, Kanaya A, Koster A, Strotmeyer ES, Goodpaster BH, Harris T, Gill TM, et al. Identifying dysglycemic states in older adults: implications of the emerging use of hemoglobin A1c. J Clin Endocrinol Metab. 2010;95:5289–5295. [PMC free article] [PubMed] [Google Scholar]
97. Grundy SM. Pre-diabetes, metabolic syndrome, and cardiovascular risk. J Am Coll Cardiol. 2012;59:635–643. [PubMed] [Google Scholar]
98. Tabák AG, Herder C, Rathmann W, Brunner EJ, Kivimäki M. Prediabetes: a high-risk state for diabetes development. Lancet. 2012;379:2279–2290. [PMC free article] [PubMed] [Google Scholar]
99. Zhang X, Gregg EW, Williamson DF, Barker LE, Thomas W, Bullard KM, Imperatore G, Williams DE, Albright AL. A1C level and future risk of diabetes: a systematic review. Diabetes Care. 2010;33:1665–1673. [PMC free article] [PubMed] [Google Scholar]
100. International Expert Committee. International Expert Committee report on the role of the A1C assay in the diagnosis of diabetes. Diabetes Care. 2009;32:1327–1334. [PMC free article] [PubMed] [Google Scholar]
101. American Diabetes Association. Diagnosis and classification of diabetes mellitus. Diabetes Care. 2009;32 Suppl 1:S62–S67. [PMC free article] [PubMed] [Google Scholar]
102. World Health Organization. Use of glycated haemoglobin (HbA1c) in the diagnosis of diabetes mellitus: Abbreviated report of a WHO consultant. Available from: http://www.who.int/diabetes/publications/report-hba1c_2011.pdf.
103. Sacks DB, Arnold M, Bakris GL, Bruns DE, Horvath AR, Kirkman MS, Lernmark A, Metzger BE, Nathan DM. Guidelines and recommendations for laboratory analysis in the diagnosis and management of diabetes mellitus. Clin Chem. 2011;57:e1–e47. [PubMed] [Google Scholar]
104. Shaw JE, d’Emden MC, Goodall I. Is Australia ready to use glycated haemoglobin for the diagnosis of diabetes? Med J Aust. 2011;195:7–8. [PubMed] [Google Scholar]
105. Davidson MB. Diagnosing diabetes with glucose criteria: worshiping a false God. Diabetes Care. 2011;34:524–526. [PMC free article] [PubMed] [Google Scholar]
106. Day A. HbA1c and diagnosis of diabetes. The test has finally come of age. Ann Clin Biochem. 2012;49:7–8. [PubMed] [Google Scholar]
107. Malkani S, Mordes JP. Implications of using hemoglobin A1C for diagnosing diabetes mellitus. Am J Med. 2011;124:395–401. [PMC free article] [PubMed] [Google Scholar]
108. Sacks DB. A1C versus glucose testing: a comparison. Diabetes Care. 2011;34:518–523. [PMC free article] [PubMed] [Google Scholar]
109. Cheng YJ, Gregg EW, Geiss LS, Imperatore G, Williams DE, Zhang X, Albright AL, Cowie CC, Klein R, Saaddine JB. Association of A1C and fasting plasma glucose levels with diabetic retinopathy prevalence in the U.S. population: Implications for diabetes diagnostic thresholds. Diabetes Care. 2009;32:2027–2032. [PMC free article] [PubMed] [Google Scholar]
110. McDonald TJ, Warren R. Diagnostic confusion? Repeat HbA1c for the diagnosis of diabetes. Diabetes Care. 2014;37:e135–e136. [PubMed] [Google Scholar]
111. Dagogo-Jack S. Pitfalls in the use of HbA:(c) as a diagnostic test: the ethnic conundrum. Nat Rev Endocrinol. 2010;6:589–593. [PubMed] [Google Scholar]
112. Ma H, Gao X, Lin HD, Hu Y, Li XM, Gao J, Zhao NQ. Glycated haemoglobin in diagnosis of diabetes mellitus and pre-diabetes among middle-aged and elderly population: Shanghai Changfeng study. Biomed Environ Sci. 2013;26:155–162. [PubMed] [Google Scholar]
113. Mukai N, Doi Y, Ninomiya T, Hata J, Hirakawa Y, Fukuhara M, Iwase M, Kiyohara Y. Cut-off values of fasting and post-load plasma glucose and HbA1c for predicting Type 2 diabetes in community-dwelling Japanese subjects: the Hisayama Study. Diabet Med. 2012;29:99–106. [PubMed] [Google Scholar]
114. Tsugawa Y, Takahashi O, Meigs JB, Davis RB, Imamura F, Fukui T, Taylor WC, Wee CC. New diabetes diagnostic threshold of hemoglobin A(1c) and the 3-year incidence of retinopathy. Diabetes. 2012;61:3280–3284. [PMC free article] [PubMed] [Google Scholar]
115. Lu ZX, Walker KZ, O’Dea K, Sikaris KA, Shaw JE. A1C for screening and diagnosis of type 2 diabetes in routine clinical practice. Diabetes Care. 2010;33:817–819. [PMC free article] [PubMed] [Google Scholar]
116. Jørgensen ME, Bjerregaard P, Borch-Johnsen K, Witte D. New diagnostic criteria for diabetes: is the change from glucose to HbA1c possible in all populations? J Clin Endocrinol Metab. 2010;95:E333–E336. [PubMed] [Google Scholar]
117. Kramer CK, Araneta MR, Barrett-Connor E. A1C and diabetes diagnosis: The Rancho Bernardo Study. Diabetes Care. 2010;33:101–103. [PMC free article] [PubMed] [Google Scholar]
118. Rathmann W, Kowall B, Tamayo T, Giani G, Holle R, Thorand B, Heier M, Huth C, Meisinger C. Hemoglobin A1c and glucose criteria identify different subjects as having type 2 diabetes in middle-aged and older populations: the KORA S4/F4 Study. Ann Med. 2012;44:170–177. [PubMed] [Google Scholar]
119. Soulimane S, Simon D, Shaw JE, Zimmet PZ, Vol S, Vistisen D, Magliano DJ, Borch-Johnsen K, Balkau B. Comparing incident diabetes as defined by fasting plasma glucose or by HbA(1c). The AusDiab, Inter99 and DESIR studies. Diabet Med. 2011;28:1311–1318. [PubMed] [Google Scholar]
120. Kawada T. Is there any ethnic difference in the prevalence of prediabetes? Am J Clin Pathol. 2012;137:500–501. [PubMed] [Google Scholar]
121. American Diabetes Association. Standards of medical care in diabetes--2010. Diabetes Care. 2010;33 Suppl 1:S11–S61. [PMC free article] [PubMed] [Google Scholar]
122. Cavagnolli G, Comerlato J, Comerlato C, Renz PB, Gross JL, Camargo JL. HbA(1c) measurement for the diagnosis of diabetes: is it enough? Diabet Med. 2011;28:31–35. [PubMed] [Google Scholar]
123. Hayes L, Hawthorne G, Unwin N. Undiagnosed diabetes in the over-60s: performance of the Association of Public Health Observatories (APHO) Diabetes Prevalence Model in a general practice. Diabet Med. 2012;29:115–120. [PubMed] [Google Scholar]
124. Costa B, Barrio F, Piñol JL, Cabré JJ, Mundet X, Sagarra R, Salas-Salvadó J, Solà-Morales O; DE-PLAN-CAT/PREDICE Research Group. Shifting from glucose diagnosis to the new HbA1c diagnosis reduces the capability of the Finnish Diabetes Risk Score (FINDRISC) to screen for glucose abnormalities within a real-life primary healthcare preventive strategy. BMC Med. 2013;11:45. [PMC free article] [PubMed] [Google Scholar]
125. Miranda JJ, Bernabe-Ortiz A, Stanojevic S, Malaga G, Gilman RH, Smeeth L. A1C as a diagnostic criteria for diabetes in low- and middle-income settings: evidence from Peru. PLoS One. 2011;6:e18069. [PMC free article] [PubMed] [Google Scholar]
126. Kharroubi AT, Darwish HM, Abu Al-Halaweh AI, Khammash UM. Evaluation of glycated hemoglobin (HbA1c) for diagnosing type 2 diabetes and prediabetes among Palestinian Arab population. PLoS One. 2014;9:e88123. [PMC free article] [PubMed] [Google Scholar]
127. HAPO Study Cooperative Research Group, Metzger BE, Lowe LP, Dyer AR, Trimble ER, Chaovarindr U, Coustan DR, Hadden DR, McCance DR, Hod M, McIntyre HD, Oats JJ, Persson B, Rogers MS, Sacks DA. Hyperglycemia and adverse pregnancy outcomes. N Engl J Med. 2008;358:1991–2002. [PubMed] [Google Scholar]
128. Metzger BE, Gabbe SG, Persson B, Buchanan TA, Catalano PA, Damm P, Dyer AR, Leiva Ad, Hod M, Kitzmiler JL, et al. International association of diabetes and pregnancy study groups recommendations on the diagnosis and classification of hyperglycemia in pregnancy. Diabetes Care. 2010;33:676–682. [PMC free article] [PubMed] [Google Scholar]
129. Diagnostic criteria and classification of hyperglycaemia first detected in pregnancy: a World Health Organization Guideline. Diabetes Res Clin Pract. 2014;103:341–363. [PubMed] [Google Scholar]
130. Roglic G, Colagiuri S. Gestational diabetes mellitus: squaring the circle. Diabetes Care. 2014;37:e143–e144. [PubMed] [Google Scholar]
131. Vandorsten JP, Dodson WC, Espeland MA, Grobman WA, Guise JM, Mercer BM, Minkoff HL, Poindexter B, Prosser LA, Sawaya GF, et al. NIH consensus development conference: diagnosing gestational diabetes mellitus. NIH Consens State Sci Statements. 2013;29:1–31. [PubMed] [Google Scholar]
132. Duran A, Sáenz S, Torrejón MJ, Bordiú E, Del Valle L, Galindo M, Perez N, Herraiz MA, Izquierdo N, Rubio MA, et al. Introduction of IADPSG criteria for the screening and diagnosis of gestational diabetes mellitus results in improved pregnancy outcomes at a lower cost in a large cohort of pregnant women: the St. Carlos Gestational Diabetes Study. Diabetes Care. 2014;37:2442–2450. [PubMed] [Google Scholar]
133. Ethridge JK, Catalano PM, Waters TP. Perinatal outcomes associated with the diagnosis of gestational diabetes made by the international association of the diabetes and pregnancy study groups criteria. Obstet Gynecol. 2014;124:571–578. [PMC free article] [PubMed] [Google Scholar]
134. Colagiuri S, Falavigna M, Agarwal MM, Boulvain M, Coetzee E, Hod M, Meltzer SJ, Metzger B, Omori Y, Rasa I, et al. Strategies for implementing the WHO diagnostic criteria and classification of hyperglycaemia first detected in pregnancy. Diabetes Res Clin Pract. 2014;103:364–372. [PubMed] [Google Scholar]
135. Trujillo J, Vigo A, Reichelt A, Duncan BB, Schmidt MI. Fasting plasma glucose to avoid a full OGTT in the diagnosis of gestational diabetes. Diabetes Res Clin Pract. 2014;105:322–326. [PubMed] [Google Scholar]
136. Sacks DB. Diagnosis of gestational diabetes mellitus: it is time for international consensus. Clin Chem. 2014;60:141–143. [PubMed] [Google Scholar]
137. Capula C, Mazza T, Vero R, Costante G. HbA1c levels in patients with gestational diabetes mellitus: Relationship with pre-pregnancy BMI and pregnancy outcome. J Endocrinol Invest. 2013;36:1038–1045. [PubMed] [Google Scholar]
138. Rajput R, Yogesh Yadav M, Nanda S. Utility of HbA1c for diagnosis of gestational diabetes mellitus. Diabetes Res Clin Pract. 2012;98:104–107. [PubMed] [Google Scholar]
139. Hughes RC, Moore MP, Gullam JE, Mohamed K, Rowan J. An early pregnancy HbA1c ≥5.9% (41 mmol/mol) is optimal for detecting diabetes and identifies women at increased risk of adverse pregnancy outcomes. Diabetes Care. 2014;37:2953–2959. [PubMed] [Google Scholar]
140. Granada C, Forbes J, Sangi-Haghpeykar H, Davidson C. Can overt diabetes mellitus be predicted by an early A1C value in gestational diabetics? J Reprod Med. 2014;59:343–347. [PubMed] [Google Scholar]
141. Starikov RS, Inman K, Chien EK, Anderson BL, Rouse DJ, Lopes V, Coustan DR. Can hemoglobin A1c in early pregnancy predict adverse pregnancy outcomes in diabetic patients? J Diabetes Complications. 2014;28:203–207. [PubMed] [Google Scholar]
142. Frayling TM. A new era in finding Type 2 diabetes genes-the unusual suspects. Diabet Med. 2007;24:696–701. [PubMed] [Google Scholar]
143. Owen KR, McCarthy MI. Genetics of type 2 diabetes. Curr Opin Genet Dev. 2007;17:239–244. [PubMed] [Google Scholar]
144. Brunetti A, Chiefari E, Foti D. Recent advances in the molecular genetics of type 2 diabetes mellitus. World J Diabetes. 2014;5:128–140. [PMC free article] [PubMed] [Google Scholar]
145. Doria A, Patti ME, Kahn CR. The emerging genetic architecture of type 2 diabetes. Cell Metab. 2008;8:186–200. [PMC free article] [PubMed] [Google Scholar]
146. Ahlqvist E, Ahluwalia TS, Groop L. Genetics of type 2 diabetes. Clin Chem. 2011;57:241–254. [PubMed] [Google Scholar]
147. Staiger H, Machicao F, Fritsche A, Häring HU. Pathomechanisms of type 2 diabetes genes. Endocr Rev. 2009;30:557–585. [PubMed] [Google Scholar]
148. Lyssenko V, Jonsson A, Almgren P, Pulizzi N, Isomaa B, Tuomi T, Berglund G, Altshuler D, Nilsson P, Groop L. Clinical risk factors, DNA variants, and the development of type 2 diabetes. N Engl J Med. 2008;359:2220–2232. [PubMed] [Google Scholar]
149. Groop L, Forsblom C, Lehtovirta M, Tuomi T, Karanko S, Nissén M, Ehrnström BO, Forsén B, Isomaa B, Snickars B, et al. Metabolic consequences of a family history of NIDDM (the Botnia study): evidence for sex-specific parental effects. Diabetes. 1996;45:1585–1593. [PubMed] [Google Scholar]
150. Weijnen CF, Rich SS, Meigs JB, Krolewski AS, Warram JH. Risk of diabetes in siblings of index cases with Type 2 diabetes: implications for genetic studies. Diabet Med. 2002;19:41–50. [PubMed] [Google Scholar]
151. Post RE, Mainous AG, King DE, Simpson KN. Dietary fiber for the treatment of type 2 diabetes mellitus: a meta-analysis. J Am Board Fam Med. 2012;25:16–23. [PubMed] [Google Scholar]
152. Zeggini E, Weedon MN, Lindgren CM, Frayling TM, Elliott KS, Lango H, Timpson NJ, Perry JR, Rayner NW, Freathy RM, et al. Replication of genome-wide association signals in UK samples reveals risk loci for type 2 diabetes. Science. 2007;316:1336–1341. [PMC free article] [PubMed] [Google Scholar]
153. Biagini Myers JM, Wang N, LeMasters GK, Bernstein DI, Epstein TG, Lindsey MA, Ericksen MB, Chakraborty R, Ryan PH, Villareal MS, et al. Genetic and environmental risk factors for childhood eczema development and allergic sensitization in the CCAAPS cohort. J Invest Dermatol. 2010;130:430–437. [PMC free article] [PubMed] [Google Scholar]
154. Scott LJ, Mohlke KL, Bonnycastle LL, Willer CJ, Li Y, Duren WL, Erdos MR, Stringham HM, Chines PS, Jackson AU, et al. A genome-wide association study of type 2 diabetes in Finns detects multiple susceptibility variants. Science. 2007;316:1341–1345. [PMC free article] [PubMed] [Google Scholar]
155. Diabetes Genetics Initiative of Broad Institute of Harvard and MIT, Lund University, and Novartis Institutes of BioMedical Research, Saxena R, Voight BF, Lyssenko V, Burtt NP, de Bakker PI, Chen H, Roix JJ, Kathiresan S, Hirschhorn JN, Daly MJ, Hughes TE, Groop L, Altshuler D, Almgren P, Florez JC, Meyer J, Ardlie K, Bengtsson Boström K, Isomaa B, Lettre G, Lindblad U, Lyon HN, Melander O, Newton-Cheh C, Nilsson P, Orho-Melander M, Råstam L, Speliotes EK, Taskinen MR, Tuomi T, Guiducci C, Berglund A, Carlson J, Gianniny L, Hackett R, Hall L, Holmkvist J, Laurila E, Sjögren M, Sterner M, Surti A, Svensson M, Svensson M, Tewhey R, Blumenstiel B, Parkin M, Defelice M, Barry R, Brodeur W, Camarata J, Chia N, Fava M, Gibbons J, Handsaker B, Healy C, Nguyen K, Gates C, Sougnez C, Gage D, Nizzari M, Gabriel SB, Chirn GW, Ma Q, Parikh H, Richardson D, Ricke D, Purcell S. Genome-wide association analysis identifies loci for type 2 diabetes and triglyceride levels. Science. 2007;316:1331–1336. [PubMed] [Google Scholar]
156. Hwang JY, Sim X, Wu Y, Liang J, Tabara Y, Hu C, Hara K, Tam CH, Cai Q, Zhao Q, et al. Genome-wide association meta-analysis identifies novel variants associated with fasting plasma glucose in East Asians. Diabetes. 2015;64:291–298. [PMC free article] [PubMed] [Google Scholar]
157. Ng MC, Shriner D, Chen BH, Li J, Chen WM, Guo X, Liu J, Bielinski SJ, Yanek LR, Nalls MA, et al. Meta-analysis of genome-wide association studies in African Americans provides insights into the genetic architecture of type 2 diabetes. PLoS Genet. 2014;10:e1004517. [PMC free article] [PubMed] [Google Scholar]
158. Dupuis J, Langenberg C, Prokopenko I, Saxena R, Soranzo N, Jackson AU, Wheeler E, Glazer NL, Bouatia-Naji N, Gloyn AL, et al. New genetic loci implicated in fasting glucose homeostasis and their impact on type 2 diabetes risk. Nat Genet. 2010;42:105–116. [PMC free article] [PubMed] [Google Scholar]
159. Horikawa Y, Oda N, Cox NJ, Li X, Orho-Melander M, Hara M, Hinokio Y, Lindner TH, Mashima H, Schwarz PE, et al. Genetic variation in the gene encoding calpain-10 is associated with type 2 diabetes mellitus. Nat Genet. 2000;26:163–175. [PubMed] [Google Scholar]
160. Tsuchiya T, Schwarz PE, Bosque-Plata LD, Geoffrey Hayes M, Dina C, Froguel P, Wayne Towers G, Fischer S, Temelkova-Kurktschiev T, Rietzsch H, et al. Association of the calpain-10 gene with type 2 diabetes in Europeans: results of pooled and meta-analyses. Mol Genet Metab. 2006;89:174–184. [PubMed] [Google Scholar]
161. Cauchi S, Meyre D, Dina C, Choquet H, Samson C, Gallina S, Balkau B, Charpentier G, Pattou F, Stetsyuk V, et al. Transcription factor TCF7L2 genetic study in the French population: expression in human beta-cells and adipose tissue and strong association with type 2 diabetes. Diabetes. 2006;55:2903–2908. [PubMed] [Google Scholar]
162. Zeggini E, McCarthy MI. TCF7L2: the biggest story in diabetes genetics since HLA? Diabetologia. 2007;50:1–4. [PMC free article] [PubMed] [Google Scholar]
163. Lyssenko V, Lupi R, Marchetti P, Del Guerra S, Orho-Melander M, Almgren P, Sjögren M, Ling C, Eriksson KF, Lethagen AL, et al. Mechanisms by which common variants in the TCF7L2 gene increase risk of type 2 diabetes. J Clin Invest. 2007;117:2155–2163. [PMC free article] [PubMed] [Google Scholar]
164. Gloyn AL, Braun M, Rorsman P. Type 2 diabetes susceptibility gene TCF7L2 and its role in beta-cell function. Diabetes. 2009;58:800–802. [PMC free article] [PubMed] [Google Scholar]
165. da Silva Xavier G, Loder MK, McDonald A, Tarasov AI, Carzaniga R, Kronenberger K, Barg S, Rutter GA. TCF7L2 regulates late events in insulin secretion from pancreatic islet beta-cells. Diabetes. 2009;58:894–905. [PMC free article] [PubMed] [Google Scholar]
166. Villareal DT, Robertson H, Bell GI, Patterson BW, Tran H, Wice B, Polonsky KS. TCF7L2 variant rs7903146 affects the risk of type 2 diabetes by modulating incretin action. Diabetes. 2010;59:479–485. [PMC free article] [PubMed] [Google Scholar]
167. Li X, Li Y, Song B, Guo S, Chu S, Jia N, Niu W. Hematopoietically-expressed homeobox gene three widely-evaluated polymorphisms and risk for diabetes: a meta-analysis. PLoS One. 2012;7:e49917. [PMC free article] [PubMed] [Google Scholar]
168. Chimienti F, Devergnas S, Pattou F, Schuit F, Garcia-Cuenca R, Vandewalle B, Kerr-Conte J, Van Lommel L, Grunwald D, Favier A, et al. In vivo expression and functional characterization of the zinc transporter ZnT8 in glucose-induced insulin secretion. J Cell Sci. 2006;119:4199–4206. [PubMed] [Google Scholar]
169. Flannick J, Thorleifsson G, Beer NL, Jacobs SB, Grarup N, Burtt NP, Mahajan A, Fuchsberger C, Atzmon G, Benediktsson R, et al. Loss-of-function mutations in SLC30A8 protect against type 2 diabetes. Nat Genet. 2014;46:357–363. [PMC free article] [PubMed] [Google Scholar]
170. SIGMA Type 2 Diabetes Consortium, Estrada K, Aukrust I, Bjørkhaug L, Burtt NP, Mercader JM, García-Ortiz H, Huerta-Chagoya A, Moreno-Macías H, Walford G, Flannick J, Williams AL, Gómez-Vázquez MJ, Fernandez-Lopez JC, Martínez-Hernández A, Jiménez-Morales S, Centeno-Cruz F, Mendoza-Caamal E, Revilla-Monsalve C, Islas-Andrade S, Córdova EJ, Soberón X, González-Villalpando ME, Henderson E, Wilkens LR, Le Marchand L, Arellano-Campos O, Ordóñez-Sánchez ML, Rodríguez-Torres M, Rodríguez-Guillén R, Riba L, Najmi LA, Jacobs SB, Fennell T, Gabriel S, Fontanillas P, Hanis CL, Lehman DM, Jenkinson CP, Abboud HE, Bell GI, Cortes ML, Boehnke M, González-Villalpando C, Orozco L, Haiman CA, Tusié-Luna T, Aguilar-Salinas CA, Altshuler D, Njølstad PR, Florez JC, MacArthur DG. Association of a low-frequency variant in HNF1A with type 2 diabetes in a Latino population. JAMA. 2014;311:2305–2314. [PMC free article] [PubMed] [Google Scholar]
171. Saxena M, Srivastava N, Banerjee M. Association of IL-6, TNF-α and IL-10 gene polymorphisms with type 2 diabetes mellitus. Mol Biol Rep. 2013;40:6271–6279. [PubMed] [Google Scholar]
172. Eirís N, González-Lara L, Santos-Juanes J, Queiro R, Coto E, Coto-Segura P. Genetic variation at IL12B, IL23R and IL23A is associated with psoriasis severity, psoriatic arthritis and type 2 diabetes mellitus. J Dermatol Sci. 2014;75:167–172. [PubMed] [Google Scholar]
173. Albert JS, Yerges-Armstrong LM, Horenstein RB, Pollin TI, Sreenivasan UT, Chai S, Blaner WS, Snitker S, O’Connell JR, Gong DW, et al. Null mutation in hormone-sensitive lipase gene and risk of type 2 diabetes. N Engl J Med. 2014;370:2307–2315. [PMC free article] [PubMed] [Google Scholar]
174. Majithia AR, Flannick J, Shahinian P, Guo M, Bray MA, Fontanillas P, Gabriel SB; GoT2D Consortium; NHGRI JHS/FHS Allelic Spectrum Project; SIGMA T2D Consortium; T2D-GENES Consortium, Rosen ED, Altshuler D. Rare variants in PPARG with decreased activity in adipocyte differentiation are associated with increased risk of type 2 diabetes. Proc Natl Acad Sci USA. 2014;111:13127–13132. [PMC free article] [PubMed] [Google Scholar]
175. Långberg EC, Seed Ahmed M, Efendic S, Gu HF, Östenson CG. Genetic association of adrenergic receptor alpha 2A with obesity and type 2 diabetes. Obesity (Silver Spring) 2013;21:1720–1725. [PubMed] [Google Scholar]
176. Karamitri A, Renault N, Clement N, Guillaume JL, Jockers R. Minireview: Toward the establishment of a link between melatonin and glucose homeostasis: association of melatonin MT2 receptor variants with type 2 diabetes. Mol Endocrinol. 2013;27:1217–1233. [PMC free article] [PubMed] [Google Scholar]
177. King DP, Zhao Y, Sangoram AM, Wilsbacher LD, Tanaka M, Antoch MP, Steeves TD, Vitaterna MH, Kornhauser JM, Lowrey PL, et al. Positional cloning of the mouse circadian clock gene. Cell. 1997;89:641–653. [PMC free article] [PubMed] [Google Scholar]
178. Turek FW, Joshu C, Kohsaka A, Lin E, Ivanova G, McDearmon E, Laposky A, Losee-Olson S, Easton A, Jensen DR, et al. Obesity and metabolic syndrome in circadian Clock mutant mice. Science. 2005;308:1043–1045. [PMC free article] [PubMed] [Google Scholar]
179. Bunger MK, Wilsbacher LD, Moran SM, Clendenin C, Radcliffe LA, Hogenesch JB, Simon MC, Takahashi JS, Bradfield CA. Mop3 is an essential component of the master circadian pacemaker in mammals. Cell. 2000;103:1009–1017. [PMC free article] [PubMed] [Google Scholar]
180. Banerjee M, Vats P. Reactive metabolites and antioxidant gene polymorphisms in type 2 diabetes mellitus. Indian J Hum Genet. 2014;20:10–19. [PMC free article] [PubMed] [Google Scholar]
181. Bener A, Darwish S, Al-Hamaq AO, Yousafzai MT, Nasralla EA. The potential impact of family history of metabolic syndrome and risk of type 2 diabetes mellitus: In a highly endogamous population. Indian J Endocrinol Metab. 2014;18:202–209. [PMC free article] [PubMed] [Google Scholar]
182. Nagamine K, Peterson P, Scott HS, Kudoh J, Minoshima S, Heino M, Krohn KJ, Lalioti MD, Mullis PE, Antonarakis SE, et al. Positional cloning of the APECED gene. Nat Genet. 1997;17:393–398. [PubMed] [Google Scholar]
183. Bennett CL, Christie J, Ramsdell F, Brunkow ME, Ferguson PJ, Whitesell L, Kelly TE, Saulsbury FT, Chance PF, Ochs HD. The immune dysregulation, polyendocrinopathy, enteropathy, X-linked syndrome (IPEX) is caused by mutations of FOXP3. Nat Genet. 2001;27:20–21. [PubMed] [Google Scholar]
184. Bordone L, Motta MC, Picard F, Robinson A, Jhala US, Apfeld J, McDonagh T, Lemieux M, McBurney M, Szilvasi A, Easlon EJ, Lin SJ, Guarente L. Sirt1 regulates insulin secretion by repressing UCP2 in pancreatic beta cells. PLoS Biol. 2006;4:e31. [PMC free article] [PubMed] [Google Scholar]
185. Sun C, Zhang F, Ge X, Yan T, Chen X, Shi X, Zhai Q. SIRT1 improves insulin sensitivity under insulin-resistant conditions by repressing PTP1B. Cell Metab. 2007;6:307–319. [PubMed] [Google Scholar]
186. Biason-Lauber A, Böni-Schnetzler M, Hubbard BP, Bouzakri K, Brunner A, Cavelti-Weder C, Keller C, Meyer-Böni M, Meier DT, Brorsson C, et al. Identification of a SIRT1 mutation in a family with type 1 diabetes. Cell Metab. 2013;17:448–455. [PMC free article] [PubMed] [Google Scholar]
187. Haller K, Kisand K, Pisarev H, Salur L, Laisk T, Nemvalts V, Uibo R. Insulin gene VNTR, CTLA-4 +49A/G and HLA-DQB1 alleles distinguish latent autoimmune diabetes in adults from type 1 diabetes and from type 2 diabetes group. Tissue Antigens. 2007;69:121–127. [PubMed] [Google Scholar]
188. Okruszko A, Szepietowska B, Wawrusiewicz-Kurylonek N, Górska M, Krętowski A, Szelachowska M. HLA-DR, HLA-DQB1 and PTPN22 gene polymorphism: association with age at onset for autoimmune diabetes. Arch Med Sci. 2012;8:874–878. [PMC free article] [PubMed] [Google Scholar]
189. Ahmadi S, Rostamzadeh J, Khosravi D, Shariati P, Shakiba N. Association of CTLA-4 gene 49A/G polymorphism with the incidence of type 1 diabetes mellitus in the Iranian Kurdish population. Pak J Biol Sci. 2013;16:1929–1935. [PubMed] [Google Scholar]
190. da Silva RC, Cunha Tavares Nde A, Moura R, Coelho A, Guimarães RL, Araújo J, Crovella S, Brandão LA, Silva Jde A. DC-SIGN polymorphisms are associated to type 1 diabetes mellitus. Immunobiology. 2014;219:859–865. [PubMed] [Google Scholar]
191. Burren OS, Guo H, Wallace C. VSEAMS: a pipeline for variant set enrichment analysis using summary GWAS data identifies IKZF3, BATF and ESRRA as key transcription factors in type 1 diabetes. Bioinformatics. 2014;30:3342–3348. [PMC free article] [PubMed] [Google Scholar]
192. Evangelou M, Smyth DJ, Fortune MD, Burren OS, Walker NM, Guo H, Onengut-Gumuscu S, Chen WM, Concannon P, Rich SS, et al. A method for gene-based pathway analysis using genomewide association study summary statistics reveals nine new type 1 diabetes associations. Genet Epidemiol. 2014;38:661–670. [PMC free article] [PubMed] [Google Scholar]
193. Tomlinson MJ, Pitsillides A, Pickin R, Mika M, Keene KL, Hou X, Mychaleckyj J, Chen WM, Concannon P, Onengut-Gumuscu S. Fine mapping and functional studies of risk variants for type 1 diabetes at chromosome 16p13.13. Diabetes. 2014;63:4360–4368. [PMC free article] [PubMed] [Google Scholar]
194. Todd JA. Etiology of type 1 diabetes. Immunity. 2010;32:457–467. [PubMed] [Google Scholar]
195. Ziegler AG, Nepom GT. Prediction and pathogenesis in type 1 diabetes. Immunity. 2010;32:468–478. [PMC free article] [PubMed] [Google Scholar]
196. UK IBD Genetics Consortium, Barrett JC, Lee JC, Lees CW, Prescott NJ, Anderson CA, Phillips A, Wesley E, Parnell K, Zhang H, Drummond H, Nimmo ER, Massey D, Blaszczyk K, Elliott T, Cotterill L, Dallal H, Lobo AJ, Mowat C, Sanderson JD, Jewell DP, Newman WG, Edwards C, Ahmad T, Mansfield JC, Satsangi J, Parkes M, Mathew CG, Donnelly P, Peltonen L, Blackwell JM, Bramon E, Brown MA, Casas JP, Corvin A, Craddock N, Deloukas P, Duncanson A, Jankowski J, Markus HS, Mathew CG, McCarthy MI, Palmer CN, Plomin R, Rautanen A, Sawcer SJ, Samani N, Trembath RC, Viswanathan AC, Wood N, Spencer CC, Barrett JC, Bellenguez C, Davison D, Freeman C, Strange A, Donnelly P, Langford C, Hunt SE, Edkins S, Gwilliam R, Blackburn H, Bumpstead SJ, Dronov S, Gillman M, Gray E, Hammond N, Jayakumar A, McCann OT, Liddle J, Perez ML, Potter SC, Ravindrarajah R, Ricketts M, Waller M, Weston P, Widaa S, Whittaker P, Deloukas P, Peltonen L, Mathew CG, Blackwell JM, Brown MA, Corvin A, McCarthy MI, Spencer CC, Attwood AP, Stephens J, Sambrook J, Ouwehand WH, McArdle WL, Ring SM, Strachan DP. Genome-wide association study of ulcerative colitis identifies three new susceptibility loci, including the HNF4A region. Nat Genet. 2009;41:1330–1334. [PMC free article] [PubMed] [Google Scholar]
197. Winkler C, Krumsiek J, Buettner F, Angermüller C, Giannopoulou EZ, Theis FJ, Ziegler AG, Bonifacio E. Feature ranking of type 1 diabetes susceptibility genes improves prediction of type 1 diabetes. Diabetologia. 2014;57:2521–2529. [PubMed] [Google Scholar]
198. Vionnet N, Stoffel M, Takeda J, Yasuda K, Bell GI, Zouali H, Lesage S, Velho G, Iris F, Passa P. Nonsense mutation in the glucokinase gene causes early-onset non-insulin-dependent diabetes mellitus. Nature. 1992;356:721–722. [PubMed] [Google Scholar]
199. Osbak KK, Colclough K, Saint-Martin C, Beer NL, Bellanné-Chantelot C, Ellard S, Gloyn AL. Update on mutations in glucokinase (GCK), which cause maturity-onset diabetes of the young, permanent neonatal diabetes, and hyperinsulinemic hypoglycemia. Hum Mutat. 2009;30:1512–1526. [PubMed] [Google Scholar]
200. Alkayyali S, Lyssenko V. Genetics of diabetes complications. Mamm Genome. 2014;25:384–400. [PubMed] [Google Scholar]
201. Fang H, Luo X, Wang Y, Liu N, Fu C, Wang H, Fang Y, Shi W, Zhang Y, Zeng C, et al. Correlation between single nucleotide polymorphisms of the ACTA2 gene and coronary artery stenosis in patients with type 2 diabetes mellitus. Exp Ther Med. 2014;7:970–976. [PMC free article] [PubMed] [Google Scholar]
202. Yamada Y, Nishida T, Ichihara S, Kato K, Fujimaki T, Oguri M, Horibe H, Yoshida T, Watanabe S, Satoh K, et al. Identification of chromosome 3q28 and ALPK1 as susceptibility loci for chronic kidney disease in Japanese individuals by a genome-wide association study. J Med Genet. 2013;50:410–418. [PubMed] [Google Scholar]
203. Shimokata S, Oguri M, Fujimaki T, Horibe H, Kato K, Yamada Y. Association between polymorphisms of the α-kinase 1 gene and type 2 diabetes mellitus in community-dwelling individuals. Biomed Rep. 2013;1:940–944. [PMC free article] [PubMed] [Google Scholar]
204. Omar AF, Silva PS, Sun JK. Genetics of diabetic retinopathy. Semin Ophthalmol. 2013;28:337–346. [PubMed] [Google Scholar]
205. Gao BB, Chen X, Timothy N, Aiello LP, Feener EP. Characterization of the vitreous proteome in diabetes without diabetic retinopathy and diabetes with proliferative diabetic retinopathy. J Proteome Res. 2008;7:2516–2525. [PubMed] [Google Scholar]
206. Fiorina P, Vergani A, Bassi R, Niewczas MA, Altintas MM, Pezzolesi MG, D’Addio F, Chin M, Tezza S, Ben Nasr M, et al. Role of podocyte B7-1 in diabetic nephropathy. J Am Soc Nephrol. 2014;25:1415–1429. [PMC free article] [PubMed] [Google Scholar]
207. Pavkov ME, Nelson RG, Knowler WC, Cheng Y, Krolewski AS, Niewczas MA. Elevation of circulating TNF receptors 1 and 2 increases the risk of end-stage renal disease in American Indians with type 2 diabetes. Kidney Int. 2015;87:812–819. [PMC free article] [PubMed] [Google Scholar]
208. Thrailkill K, Bunn RC, Lumpkin C, Wahl E, Cockrell G, Morris L, Kahn CR, Fowlkes J, Nyman JS. Loss of insulin receptor in osteoprogenitor cells impairs structural strength of bone. J Diabetes Res. 2014;2014:703589. [PMC free article] [PubMed] [Google Scholar]
209. Vernochet C, Damilano F, Mourier A, Bezy O, Mori MA, Smyth G, Rosenzweig A, Larsson NG, Kahn CR. Adipose tissue mitochondrial dysfunction triggers a lipodystrophic syndrome with insulin resistance, hepatosteatosis, and cardiovascular complications. FASEB J. 2014;28:4408–4419. [PMC free article] [PubMed] [Google Scholar]
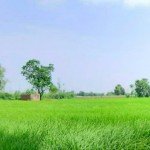