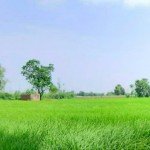
Abstract
Coronavirus disease 2019 (COVID-19) originated in the city of Wuhan, Hubei Province, Central China, and has spread quickly to 72 countries to date. COVID-19 is caused by a novel coronavirus, named severe acute respiratory syndrome coronavirus 2 (SARS-CoV-2) [previously provisionally known as 2019 novel coronavirus (2019-nCoV)]. At present, the newly identified SARS-CoV-2 has caused a large number of deaths with tens of thousands of confirmed cases worldwide, posing a serious threat to public health. However, there are no clinically approved vaccines or specific therapeutic drugs available for COVID-19. Intensive research on the newly emerged SARS-CoV-2 is urgently needed to elucidate the pathogenic mechanisms and epidemiological characteristics and to identify potential drug targets, which will contribute to the development of effective prevention and treatment strategies. Hence, this review will focus on recent progress regarding the structure of SARS-CoV-2 and the characteristics of COVID-19, such as the aetiology, pathogenesis and epidemiological characteristics.
Keywords: COVID-19, SARS-CoV-2, 2019-nCoV, Coronavirus, Pneumonia
1. Introduction
Coronaviruses (CoVs) belong to the subfamily Orthocoronavirinae in the family Coronaviridae, Order Nidovirales. There are four genera within the subfamily Orthocoronavirinae, namely Alphacoronavirus (α-CoV), Betacoronavirus (β-CoV), Gammacoronavirus (γ-CoV) and Deltacoronavirus (δ-CoV) [1,2]. The CoV genome is an enveloped, positive-sense, single-stranded RNA with a size varying between 26 kb and 32 kb, the largest genome of known RNA viruses. Both α- and β-CoV genera are known to infect mammals, whilst δ- and γ-CoVs infect birds. Two recent outbreaks of viral pneumonia caused by β-CoVs are severe acute respiratory syndrome (SARS) and Middle East respiratory syndrome (MERS). In 2002, an outbreak of SARS was first reported in China and then spread quickly worldwide, resulting in hundreds of deaths with an 11% mortality rate [3,4]. In 2012, MERS first emerged in Saudi Arabia and subsequently spread to other countries, with a fatality rate of 37% [5], [6], [7]. In both of these epidemics, the viruses likely originated from bats and then infected humans through other intermediate animal hosts, e.g. the civet (Paguma larvata) for SARS-CoV and the camel for MERS-CoV [8], [9], [10].
Beginning in December 2019, a number of patients with pneumonia of unknown aetiology emerged in Wuhan City, Hubei Province, Central China. Genome sequencing has demonstrated that this pneumonia, named coronavirus disease 2019 (COVID-19), is caused by a novel CoV, namely severe acute respiratory syndrome coronavirus 2 (SARS-CoV-2), previously known as 2019 novel coronavirus (2019-nCoV) [11], [12], [13]. Like SARS-CoV and MERS-CoV, this newly emerged SARS-CoV-2 virus belongs to the B lineage of the β-CoVs.
To date, COVID-19 has spread rapidly in 72 countries, causing >90 000 confirmed cases and over 2946 deaths as of 3 March 2020. Considering the global threat, the World Health Organization (WHO) has declared COVID-19 a public health emergency of international concern (PHEIC). However, there are no vaccines against SARS-CoV-2 or specific therapeutic drugs for this communicable disease. Thus, a better understanding of SARS-CoV-2 is essential for exploring effective vaccines and drugs. In this review, we summarise recent progress in SARS-CoV-2 to provide a framework for the prevention and treatment of COVID-19.
2. Structure of SARS-CoV-2
The SARS-CoV-2 genome (30 kb in size) encodes a large, non-structural polyprotein (ORF1a/b) that is further proteolytically cleaved to generate 15/16 proteins, 4 structural proteins and 5 accessory proteins (ORF3a, ORF6, ORF7, ORF8 and ORF9) [14], [15], [16] (Fig. 1 ). The four structural proteins consist of the spike (S) surface glycoprotein, the membrane (M) protein, the envelope (E) protein and the nucleocapsid (N) protein, which are essential for SARS-CoV-2 assembly and infection. The spike surface glycoprotein plays a key role in its attachment to host cells and can be further cleaved by host proteases into an N-terminal S1 subunit and a membrane-bound C-terminal S2 region [17]. Binding of the S1 subunit to a host receptor can destabilise the prefusion trimer, leading to shedding of the S1 subunit and transition of the S2 subunit into a highly stable postfusion conformation [18]. In order to engage a host receptor, the receptor-binding domain (RBD) of the S1 subunit undergoes hinge-like conformational movements, which transiently hide or expose the determinants of receptor binding [19,20]. These two states of the S1 subunit can be regarded as the ‘down’ conformation and the ‘up’ conformation. The former represents an inaccessible state of the receptor, whereas the latter corresponds to an accessible state [19,21]. Therefore, understanding the structure and function of the spike protein can help to develop monoclonal antibody drugs and to guide the design and development of vaccines.
Structure and genome of severe acute respiratory syndrome coronavirus 2 (SARS-CoV-2). (A) There are four structural proteins as follows: spike (S) surface glycoprotein (purple); membrane (M) protein (orange); nucleocapsid (N) protein (blue); and envelope ...
3. Etiology and pathogenesis of COVID-19
SARS-CoV-2 is the seventh member of the family of CoVs that infect humans. Four human CoVs (HCoV-229E, HCoV-NL63, HCoV-OC43 and HCoV-HKU1) are able to cause a wide range of upper respiratory tract infections (common cold), whereas SARS-CoV and MERS-CoV are responsible for atypical pneumonia. The causes of different infection sites are likely related to the presence of dipeptidyl peptidase 4 (DPP4) and angiotensin-converting enzyme 2 (ACE2) in the lower respiratory tract, which are the major human receptors for the surface spike (S) glycoprotein of MERS-CoV and SARS-CoV, respectively [22], [23], [24]. The genetic sequence of SARS-CoV-2 is ≥70% similar to that SARS-CoV, and SARS-CoV-2 is capable of using the same cell entry receptor (ACE2) as SARS-CoV to infect humans [25,26]. However, there are more differences in the key S proteins that the viruses use to interact with host cells. SARS-CoV-2 spike binds to human ACE2 with approximately 10–20-fold higher affinity than the SARS-CoV spike [19], making it easier to spread from human to human.
Upon entry into alveolar epithelial cells, SARS-CoV-2 replicates rapidly and triggers a strong immune response, resulting in cytokine storm syndromes and pulmonary tissue damage. Cytokine storm syndromes, also known as hypercytokinaemia, are a group of disorders characterised by the uncontrolled production of pro-inflammatory cytokines and are important causes of acute respiratory distress syndrome (ARDS) and multiple organ failure [27], [28], [29]. Analysis of the first 99 confirmed cases of SARS-CoV-2 infection revealed that cytokine storm syndromes occurred in patients with severe COVID-19; 17 patients (17%) had ARDS, among whom 11 (11%) deteriorated within a short period of time and died of multiple organ failure [30]. In addition, the numbers of total T-cells, CD4+ T-cells and CD8+ T-cells are decreased in patients with SARS-CoV-2 infection, and the surviving T-cells are functionally exhausted [31], suggesting a decreased immune function in SARS-CoV-2-infected patients. ARDS, decreased immune function and secondary infection further worsens respiratory failure.
4. Epidemiological characteristics of SARS-CoV-2
4.1. Progress and current status of the epidemic
Since December 2019, multiple cases of patients with pneumonia of unknown cause were successively reported from the Chinese city of Wuhan. They shared a history of exposure to the Huanan seafood market in Wuhan and the disease was been confirmed to be an acute respiratory infection caused by a novel CoV. To date, the number of patients without a history of exposure to the Huanan seafood market is significantly increased. In addition, some cases without a history of travel to Wuhan have emerged [32]. These early phenomena suggest the possibility of human-to-human transmission.
At present, the National Health Commission of the People's Republic of China has recorded a total of 80 304 confirmed cases of pneumonia with SARS-CoV-2 infection from 34 provinces (districts and cities). These included 6806 serious illnesses and 2946 deaths. By 24:00 on 3 March 2020, 2308 suspected cases were recorded, 664 899 with close contacts to the confirmed patients have been tracked, 7650 people were released from medical observation that day, and 40 651 people were still undergoing medical observation. A total of 137 confirmed cases were reported from Macao Special Administrative Region (SAR) (n = 10), Hong Kong SAR (n = 93) and Taiwan (Republic of China) (n = 34). In addition to China, 46 different countries from Western Pacific Asia, Northern America, Eastern Mediterranean, Southeast Asia, African and Europe have reported confirmed cases of this disease, making a total tally of confirmed cases of 90 870 worldwide.
4.2. Origin of SARS-CoV-2
The initial source of SARS-CoV-2 is still unknown, although the first cases were linked to the Huanan seafood market in Wuhan city. Besides seafood, it is reported on social media that some wild animals including birds, snakes, marmots and bats were sold at the Huanan seafood market. It has been reported that environmental samples obtained from the marketplace have come back positive for the novel CoV, but the specific animal has not been identified [33]. More recently, several studies have suggested that bats may be the potential natural host of SARS-CoV-2 [26,34,35]. The whole genome-wide nucleotide sequence of SARS-CoV-2 is 96% identical to a bat CoV [26]. Importantly, SARS-CoV-2 has been isolated from pangolins and it was found that the isolated pangolin CoV genomes have ~85.5–92.4% similarity to SARS-CoV-2 [36], suggesting that pangolin may be a potential intermediate host for SARS-CoV-2. However, whether SARS-CoV-2 is transmitted directly from bats or by an intermediate host requires further confirmation. Learning from the role of camels in MERS and civets in SARS, hunting for the source of SARS-CoV-2 will help determine zoonotic transmission patterns and stem the ongoing outbreak.
4.3. Transmission route of SARS-CoV-2
The novel CoV can be transmitted between humans via respiratory droplets. Notably, the respiratory tract is probably not the only route of transmission. Close contact is also a source of transmission of SARS-CoV-2. For example, SARS-CoV-2 can be transmitted through direct or indirect contact with mucous membranes in the eyes, mouth or nose [37], [38], [39]. There is also a possibility of aerosol transmission in a relatively closed environment with continuous exposure to high concentrations of aerosol. Moreover, it has been reported that COVID-19 patients have some gastrointestinal symptoms, including diarrhoea, nausea and vomiting [40,41]. A recent study showed that the enteric symptoms of COVID-19 pneumonia are associated with invaded ACE2-expressing enterocytes [42], suggesting that the digestive tract is a potential route of SARS-CoV-2 infection besides the respiratory tract. However, additional studies are required to test this possibility. In addition, whether transmission of SARS-CoV-2 can occur via breast milk or vertically from mother to infant has not been determined.
4.4. Susceptible population
All populations are generally susceptible to SARS-CoV-2. The elderly and people with underlying diseases or low immune function are more likely to become severe cases [30,43]. In addition, pregnant women and newborns infected with SARS-CoV-2 are also prone to develop severe pneumonia [44,45]. Thus, these vulnerable patients should be considered as a focus in the prevention and management of SARS-CoV-2 infection.
4.5. Incubation period
The mean incubation period of SARS-CoV-2 is estimated to be 3–7 days (range, 2–14 days) [46,47], indicating a long transmission period of SARS-CoV-2. It is estimated that SARS-CoV-2 latency is consistent with those of other known human CoVs, including non-SARS human CoVs (mean 3 days, range 2–5 days) [48], SARS-CoV (mean 5 days, range 2–14 days) [49] and MERS-CoV (mean 5.7 days, range 2–14 days) [50]. Moreover, it has been reported that asymptomatic COVID-19 patients during their incubation periods can effectively transmit SARS-CoV-2 [51,52], which is different from SARS-CoV because most SARS-CoV cases are infected by ‘superspreaders’ and SARS-CoV cases cannot infect susceptible persons during the incubation period [53]. Taken together, these data fully support the current period of active monitoring recommended by the WHO of 14 days.
4.6. Basic reproduction number (R0) of SARS-CoV-2
The basic reproduction number is a very important threshold related to the transmissibility of the virus, which is usually expressed as R nought (R0). The R0 can be implicitly defined as the average number of secondary infections produced by an infectious person. It is generally believed that if R0 is >1, the number of infected cases will increase exponentially and cause an epidemic or even a pandemic. Liu et al. reviewed the R0 of SARS-CoV-2 and found that the estimates ranged from 1.4–6.49, with a mean of 3.28 [54], which is higher than that of SARS-CoV (R0 of 2~5).
4.7. Clinical presentation
At the onset of the disease, the main manifestations of COVID-19 are fatigue, fever, dry cough, myalgia and dyspnoea, with less common symptoms being nasal congestion, headache, runny nose, sore throat, vomiting and diarrhoea. Severe patients often have dyspnoea and/or hypoxemia 1 week after onset, after which septic shock, ARDS, difficult-to-correct metabolic acidosis, and coagulation dysfunction develop rapidly. Of note, severe and critical patients can also only present with a low fever, or even no obvious fever, and mild patients show only low fever, mild fatigue and no pneumonia [40,43]. These asymptomatic or mild cases can also spread SARS-CoV-2 between humans.
4.8. Pathological characteristics
Recently, Xu et al. reported the pathological features of the first patient known to have died from SARS-CoV-2 infection [55]. Biopsy samples were obtained from lung tissue of the patient and it was found that the pathological features of COVID-19 are related to ARDS. For example, evident desquamation of pneumocytes and hyaline membrane formation were seen in the lung tissue, indicating ARDS. Moreover, interstitial mononuclear inflammatory infiltration was observed in lung tissue. Multinucleated giant cells with atypical enlarged pneumocytes characterised by large nuclei, prominent nucleoli and amphophilic granular cytoplasm were observed in the intra-alveolar spaces, suggesting viral cytopathic-like changes [55]. These pathological characteristics of COVID-19 are highly similar to those seen in SARS-CoV and MERS-CoV infection [56,57]. Taken together, understanding the pathological characteristics of this severe case of COVID-19 could help to provide new insights into the pathogenesis of SARS-CoV-2-infected pneumonia, which may help physicians to formulate a timely strategy for the treatment of similar severe patients and to decrease mortality.
4.9. Computed tomography (CT) imaging characteristics
CT is often found to be positive when patients with SARS-CoV-2 develop a persistent cough, fever and unexplained fatigue. Typical CT presentations of COVID-19 patients include bilateral pulmonary parenchymal ground-glass opacity, pulmonary consolidation and nodules, bilateral diffuse distribution, sometimes with a rounded morphology, and a peripheral lung distribution [58,59]. In the early course of disease, chest images show multiple small patchy shadows and interstitial changes, which are evident in the lung periphery. Severe cases can spread to the bronchi with progress of the disease, gradually spread to the whole lung, and with infrequent interlobar pleural thickening and pleural effusion.
4.10. Detection of SARS-CoV-2
Rapid and accurate detection of SARS-CoV-2 is essential to control the outbreak of COVID-19. Nucleic acid detection is a major method of laboratory diagnosis. Reverse transcription quantitative PCR (RT-qPCR) is a molecular biological diagnosis technology based on nucleic acid sequences. The complete SARS-CoV-2 genome sequences are available in GenBank. Thus, the nucleic acid of SARS-CoV-2 can be detected by RT-qPCR or by viral gene sequencing of nasopharyngeal and oropharyngeal swabs, stool, sputum or blood samples [60,61]. However, collection of these specimen types by healthcare workers requires close contact with patients, which poses a risk of spreading the virus to healthcare workers. Moreover, collection of nasopharyngeal or oropharyngeal specimens may cause bleeding, especially in patients with thrombocytopenia [32]. Importantly, To et al. found that SARS-CoV-2 could be effectively detected in the saliva specimens of infected patients [62], suggesting that saliva is a promising non-invasive specimen type for diagnosis, monitoring and infection control of COVID-19 patients.
Besides RT-qPCR, Zhang et al. described a protocol using the CRISPR-based SHERLOCK (Specific High-sensitivity Enzymatic Reporter UnLOCKing) technique for the detection of SARS-CoV-2 [63]. Using synthetic SARS-CoV-2 virus RNA fragments, the authors found that this technique is able to consistently detect target sequences of SARS-CoV-2 in a range between 20 and 200 aM (10–100 copies per microlitre of input). This test can be read out using a dipstick in <1 h, without requiring elaborate instrumentation [63]. Compared with RT-qPCR, the SHERLOCK technique is more accurate and the detection time is reduced by one-half. Thus, use of the SHERLOCK technique for the detection of SARS-CoV-2 in clinical patient samples is expected.
5. Treatments
Suspected and confirmed cases should be treated in designated hospitals with effective isolation and protective conditions. Suspected cases should be treated in a single room and isolated, and confirmed cases can be treated in the same ward. Moreover, critical cases need to be admitted to the intensive care unit as soon as possible.
5.1. General treatments
General treatment strategies include bed rest and supportive treatments, ensuring sufficient energy intake, maintaining a constant internal environment (water, electrolytes and other internal environment factors) and monitoring vital signs (heart rate, pulse, blood pressure, oxygen saturation, respiratory rate, etc.).
5.2. Antiviral therapy
5.2.1. Interferon-alpha (IFNα)
IFNα is a member of the family of type I IFNs that plays an important role in host resistance to viral infection. IFNα suppresses viral infection by directly interfering with replication of the virus and by promoting both innate and adaptive immune responses. In vitro experiments showed that IFNα effectively inhibits the replication of SARS-CoV [64,65]. It has also been reported that cynomolgus monkeys are protected from infection with SARS CoV by treatment with IFNα [66]. Moreover, the therapeutic benefit of synthetic recombinant IFNα for patients with SARS was demonstrated in a pilot clinical trial [67]. Thus, IFNα should be considered a candidate drug for COVID-19 therapy.
5.2.2. Lopinavir/ritonavir (Kaletra)
Lopinavir/ritonavir was first known as a protease inhibitor that interferes with the replication and synthesis of human immunodeficiency virus (HIV), leading to the production of immature, non-infectious virus particles [68,69]. It has been reported that ritonavir and lopinavir can bind to the endopeptidase C30 of SARS-CoV-2 protease as evaluated by molecular models [70]. This suggests that lopinavir/ritonavir may exert an antiviral effect by inhibiting protein synthesis of SARS-CoV-2. In addition, several lines of evidence showed that treatment with lopinavir/ritonavir alone or in combination with other antiviral drugs was shown to improve the outcome of severe patients with SARS or MERS by ameliorating ARDS [71], [72], [73]. Given that SARS-CoV-2 is similar to these two viruses, lopinavir/ritonavir may have a beneficial effect on COVID-19. Future studies are needed to test this possibility.
5.2.3. Ribavirin
Ribavirin is a nucleoside analogue with broad antiviral activity. It can prevent the replication of RNA and DNA viruses by suppressing the activity of inosine monophosphate dehydrogenase, which is required for the synthesis of guanosine triphosphate (GTP). Ribavirin was widely used to treat SARS patients with or without concomitant use of steroids during the outbreak of SARS in Hong Kong [74], [75], [76]. Thus, ribavirin could be considered as a treatment option for COVID-19 patients.
5.2.4. Chloroquine
Chloroquine is a widely used antimalarial and autoimmune disease drug. Recently, chloroquine has been reported as a potential broad-spectrum antiviral drug [77,78]. Wang et al. found that chloroquine effectively suppresses the recently emerged novel CoV (SARS-CoV-2) in vitro [79]. Chloroquine is a cheap and safe drug that has been used for more than 70 years and thus it is potentially clinically applicable against COVID-19.
5.2.5. Arbidol (umifenovir)
Arbidol is an antiviral drug against influenza infection that is widely used in Russia and China. Arbidol and arbidol mesylate were shown to have a potent inhibitory effect in reducing the reproduction of SARS-CoV in vitro [80]. Low-level evidence including a retrospective cohort study, case reports and case series revealed that arbidol alone or combined with antiviral drugs produced certain benefits in the treatment of COVID-19 pneumonia [81], [82], [83]. Currently, many randomised clinical controlled trials are being carried out studying the efficacy of Arbidol on COVID-19 pneumonia in China.
5.2.6. Remdesivir
The nucleoside analogue remdesivir (GS-5734) was reported to inhibit SARS-CoV and MERS-CoV in vivo [84,85]. More recently, an in vitro study showed that remdesivir potently blocked SARS-CoV-2 infection at low-micromolar concentrations and showed a high selectivity index [79]. In addition, the first case of SARS-CoV-2 infection in the USA was treated with intravenous remdesivir when the patient's condition deteriorated [41]. Although remdesivir has some benefits for the treatment of COVID-19 pneumonia, randomised controlled trials are still required to determine its efficacy and safety.
Taken together, these antiviral drugs may be promising treatment options for the treatment of COVID-19. However, there are also a few points worth noting here: (i) the potential interaction of these antiviral drugs with other therapeutic drugs should be considered; (ii) adverse reactions caused by lopinavir/ritonavir, such as diarrhoea, nausea, vomiting and liver damage, should be also considered; (iii) it is not recommended to use three or more antiviral drugs at the same time, and the use of related drugs should be stopped when there are intolerable side effects; and (iv) further evaluation of the efficacy of current antiviral drugs in clinical applications is needed.
5.3. Cellular therapy
5.3.1. Natural killer (NK) cells
NK cells are important immune cells necessary for defence against microbe-infected, stressed or malignant cells. Human NK cells lyse antibody-coated virus-infected cells via the process of antibody-dependent cellular cytotoxicity (ADCC) [86]. In this way, NK cells are specific to almost all virus-infected cells. Several studies have shown that NK cells can exert antiviral activity by mediating ADCC against SARS-CoV, herpes simplex virus type 1 (HSV-1), cytomegalovirus and HIV [87], [88], [89]. Umbilical cord blood is a promising source of allogeneic NK cells. Recently, Sorrento and Celularity have announced the launch of a clinical collaboration aimed at extending the use of CYNK-001, an allogeneic, off-the-shelf, umbilical cord blood-derived NK cell therapy, to the treatment of the newly emerged SARS-CoV-2 infection [90]. Whilst developing new drugs, new vaccines or clinical trials of old drugs, carrying out NK cell therapy to enhance immunity is currently a very feasible strategy for the treatment and prevention of COVID-19 pneumonia.
5.3.2. Mesenchymal stem cells (MSCs)
It is well known that MSCs have strong anti-inflammatory and immunomodulatory functions. Umbilical cord blood and placenta are good sources of MSCs. Numerous studies have shown that treatment with MSCs can ameliorate acute/chronic lung injury and ARDS by suppressing the infiltration of immune cells to pulmonary tissues and pro-inflammatory cytokine secretion [91], [92], [93], [94]. In addition, MSCs contribute to reducing lung fibrosis and enhancing tissue repair [95,96]. Besides routine antiviral treatment, it is important to treat cytokine storm syndromes, ARDS and acute lung injury in patients with severe COVID-19 to prevent progression of the disease and to reduce mortality. Thus, MSCs may be a promising therapeutic option.
5.4. Immunotherapy
5.4.1. Convalescent plasma therapy
Antiviral antibodies (IgG, IgA, IgM, IgE and IgD) found in convalescent plasma from recovered patients can effectively treat patients with viral infections. Convalescent plasma therapy has been widely used in infectious diseases such as poliomyelitis, influenza A (H5N1) and Ebola [97], [98], [99]. In addition, such passive immunisation can also be achieved by using convalescent plasma from patients with SARS-CoV infection. It has been reported that a small number of SARS-CoV-infected patients in Taiwan and Hong Kong received treatment with convalescent plasma during the early course of the disease with certain clinical benefits, including a reduction of plasma viral load from ~105 copies/mL to undetectable levels 24 h after plasma transfusion [100], [101], [102]. Thus, convalescent plasma could, theoretically, be a promising option for the treatment and prevention of SARS-CoV-2 infection, although this has not been tested clinically.
5.4.2. Monoclonal antibodies
Remission of the SARS-CoV-2 epidemic may depend on the development of monoclonal antibodies. Previous studies have identified a number of effective monoclonal antibodies that target the SARS-CoV spike protein to prevent the virus from entering host cells [103], [104], [105]. The 193-amino acid (residues 318–510) receptor-binding domain (RBD) of the spike protein is the key target of neutralising monoclonal antibodies [106]. The SARS-CoV neutralising monoclonal antibodies CR3014 and CR3022 were found to bind non-competitively to the SARS-CoV RBD and neutralised the virus in a synergistic manner [104]. A recent study showed that CR3022 could combine effectively with SARS-CoV-2 RBD (KD of 6.3 nM) [107]. Moreover, the epitope of CR3022 does not overlap with the binding site of ACE2 in the SARS-CoV-2 RBD [107]. Thus, CR3022 could be a promising therapeutic candidate, alone or in combination with other neutralising monoclonal antibodies, for the treatment of COVID-19 pneumonia.
5.5. Chinese medicine
Glycyrrhizin, an active component of liquorice roots used in Chinese medicine, could effectively inhibit the replication of SARS-associated CoV in vitro [108,109]. Furthermore, high doses of glycyrrhizin have been used in clinical trials and the compound was reported to be clinically effective for the treatment of SARS at that time [110,111]. Recently, glycyrrhizin was predicted to have the ability to bind ACE2 with potential anti-COVID-19 effects [112]. Hesperetin, a well-known traditional Chinese medicine, is a natural predominant flavonoid found in citrus fruits. Hesperetin dose-dependently suppresses the cleavage activity of the 3C-like protease (3CLpro) of SARS-CoV in cell-free and cell-based assays [113]. Hesperetin was also reported to have the potential to inhibit ACE2 and therefore block infection with SARS-CoV-2 [112]. Baicalin, another traditional Chinese herbal medicine, is a flavone isolated from Scutellaria baicalensis. It has been shown that baicalin has antiviral activity against 10 clinical isolates of SARS-CoV by neutralisation tests [114]. In addition, quercetin is a plant flavone that is widely used in traditional Chinese medicine and botanical medicine. Quercetin was reported to exert antiviral effects by inhibiting the 3CLpro of SARS-CoV [115] and blocking the entry of SARS-CoV into host cells [116]. Therefore, these studies suggest that Chinese medicine also plays a key role in the prevention and treatment of COVID-19 pneumonia.
6. Conclusion and future directions
SARS-CoV first appeared in 2002 and rapidly spread to 32 countries and regions, after which the world then experienced the outbreak of MERS-CoV in 2012. Recently, the newly emerged SARS-CoV-2 is undoubtedly a warning. It has been confirmed that SARS-CoV-2 enters lung cells by binding to ACE2. Besides pulmonary tissue, ACE2 is also highly expressed in other tissues including bile duct, liver, gastrointestinal organs (small intestine, duodenum), oesophagus, testis and kidney [42,[117], [118], [119]]. Thus, these organs may also be damaged by SARS-CoV-2. Currently, SARS-CoV-2 has spread rapidly in multiple countries, caused severe illness and sustained human-to-human transmission, making it a concerning and serious public-health threat. Considering the global threat to health caused by SARS-CoV-2, effective prevention and treatment of COVID-19 pneumonia will be urgently needed. However, the development of drugs for pathogenic SARS-CoV-2 is still a major problem for humans, and there are currently no officially approved drugs to treat COVID-19. In view of the epidemiological characteristics of SARS-CoV-2, it is crucial to interrupt the spread of the virus through epidemic prevention and control methods, such as isolating infected patients and controlling the source of infection. At the same time, wild animal trafficking must be banned to reduce the spread of CoVs. In addition, it is necessary to make full use of the currently limited evidence for therapeutic drugs to develop strategies of drug application to prevent and control the transmission of SARS-CoV-2. It has been confirmed that the genome sequence of SARS-CoV-2 shares high identity with that of bat and human SARS-CoVs. A number of antiviral drugs and treatment methods for SARS-CoV infection have also been considered for the treatment and prevention of COVID-19 pneumonia.
We have been warned three times in recent years. CoVs are highly vulnerable to public-health intervention strategies, but the increasing incidence of CoV emergence in livestock animal populations and the identification of novel CoVs in reservoir species do not support this view. Therefore, in addition to developing new drugs and clinical trials of old drugs, the design and development of vaccines for SARS-CoV-2 is also needed. Lessons from SARS-CoV and MERS-CoV suggest that SARS-CoV-2 research should focus on the establishment of animal models that recapitulate the various aspects of human disease and determinants of vaccine safety and efficacy.
Acknowledgments
Funding: This work was supported by the Natural Science Foundation of China [nos. 81570408 and 81770461].
Competing interests: None declared.
Ethical approval: Not required.
Notes
Editor: Jean-Marc Rolain
Article information
Int J Antimicrob Agents. 2020 May; 55(5): 105951.
Published online 2020 Mar 29. doi: 10.1016/j.ijantimicag.2020.105951
PMCID: PMC7139247
PMID: 32234466
Heng Li,a,1 Shang-Ming Liu,a,1 Xiao-Hua Yu,b,1 Shi-Lin Tang,a,⁎⁎ and Chao-Ke Tanga,⁎
aInstitute of Cardiovascular Disease, Key Laboratory for Arteriosclerology of Hunan Province, Department of Intensive Care Unit, the First Affiliated Hospital of University of South China, Medical Research Experiment Center, Hunan Province Cooperative Innovation Center for Molecular Target New Drug Study, Hengyang Medical College, University of South China, Hengyang, Hunan 421001, China
bInstitute of Clinical Medicine, The Second Affiliated Hospital of Hainan Medical University, Haikou, Hainan 460106, China
Shi-Lin Tang: moc.qq@328657682; Chao-Ke Tang: moc.qq@ekoahcgnat
⁎Corresponding authors. Present addresses: Institute of Cardiovascular Disease, University of South China, Hengyang, Hunan 421001, China. moc.qq@ekoahcgnat
⁎⁎Department of Intensive Care Unit, the First Affiliated Hospital of University of South China, Hengyang, Hunan 421001, China. moc.qq@328657682
1These three authors contributed equally to this work.
Received 2020 Mar 6; Accepted 2020 Mar 19.
Copyright © 2020 Elsevier B.V. and International Society of Chemotherapy. All rights reserved.
Since January 2020 Elsevier has created a COVID-19 resource centre with free information in English and Mandarin on the novel coronavirus COVID-19. The COVID-19 resource centre is hosted on Elsevier Connect, the company's public news and information website. Elsevier hereby grants permission to make all its COVID-19-related research that is available on the COVID-19 resource centre - including this research content - immediately available in PubMed Central and other publicly funded repositories, such as the WHO COVID database with rights for unrestricted research re-use and analyses in any form or by any means with acknowledgement of the original source. These permissions are granted for free by Elsevier for as long as the COVID-19 resource centre remains active.
This article has been cited by other articles in PMC.
References
1. Banerjee A, Kulcsar K, Misra V, Frieman M, Mossman K. Bats and coronaviruses. Viruses. 2019;11 doi: 10.3390/v11010041. pii: E41. [PMC free article] [PubMed] [CrossRef] [Google Scholar]
2. Yang D, Leibowitz JL. The structure and functions of coronavirus genomic 3′ and 5′ ends. Virus Res. 2015;206:120–133. doi: 10.1016/j.virusres.2015.02.025. [PMC free article] [PubMed] [CrossRef] [Google Scholar]
3. Song Z, Xu Y, Bao L, Zhang L, Yu P, Qu Y. From SARS to MERS, thrusting coronaviruses into the spotlight. Viruses. 2019;11 doi: 10.3390/v11010059. pii: E59. [PMC free article] [PubMed] [CrossRef] [Google Scholar]
4. Graham RL, Donaldson EF, Baric RS. A decade after SARS: strategies for controlling emerging coronaviruses. Nat Rev Microbiol. 2013;11:836–848. doi: 10.1038/nrmicro3143. [PMC free article] [PubMed] [CrossRef] [Google Scholar]
5. Zumla A, Hui DS, Perlman S. Middle East respiratory syndrome. Lancet. 2015;386:995–1007. doi: 10.1016/S0140-6736(15)60454-8. [PMC free article] [PubMed] [CrossRef] [Google Scholar]
6. Hui DS, Azhar EI, Kim YJ, Memish ZA, Oh MD, Zumla A. Middle East respiratory syndrome coronavirus: risk factors and determinants of primary, household, and nosocomial transmission. Lancet Infect Dis. 2018;18:e217–e227. doi: 10.1016/S1473-3099(18)30127-0. [PMC free article] [PubMed] [CrossRef] [Google Scholar]
7. Su S, Wong G, Liu Y, Gao GF, Li S, Bi Y. MERS in South Korea and China: a potential outbreak threat? Lancet. 2015;385:2349–2350. doi: 10.1016/S0140-6736(15)60859-5. [PMC free article] [PubMed] [CrossRef] [Google Scholar]
8. Reusken CB, Haagmans BL, Müller MA, Gutierrez C, Godeke GJ, Meyer B. Middle East respiratory syndrome coronavirus neutralising serum antibodies in dromedary camels: a comparative serological study. Lancet Infect Dis. 2013;13:859–866. doi: 10.1016/S1473-3099(13)70164-6. [PMC free article] [PubMed] [CrossRef] [Google Scholar]
9. de Wit E, van Doremalen N, Falzarano D, Munster VJ. SARS and MERS: recent insights into emerging coronaviruses. Nat Rev Microbiol. 2016;14:523–534. doi: 10.1038/nrmicro.2016.81. [PMC free article] [PubMed] [CrossRef] [Google Scholar]
10. Lu G, Wang Q, Gao GF. Bat-to-human: spike features determining ‘host jump’ of coronaviruses SARS-CoV, MERS-CoV, and beyond. Trends Microbiol. 2015;23:468–478. doi: 10.1016/j.tim.2015.06.003. [PMC free article] [PubMed] [CrossRef] [Google Scholar]
11. Xu X, Chen P, Wang J, Feng J, Zhou H, Li X. Evolution of the novel coronavirus from the ongoing Wuhan outbreak and modeling of its spike protein for risk of human transmission. Sci China Life Sci. 2020;63:457–460. doi: 10.1007/s11427-020-1637-5. [PMC free article] [PubMed] [CrossRef] [Google Scholar]
12. Wong JEL, Leo YS, Tan CC. COVID-19 in Singapore—current experience: critical global issues that require attention and action. JAMA. 2020 Feb 20 doi: 10.1001/jama.2020.2467. [Epub ahead of print] [PubMed] [CrossRef] [Google Scholar]
13. Li Q, Guan X, Wu P, Wang X, Zhou L, Tong Y. Early transmission dynamics in Wuhan, China, of novel coronavirus-infected pneumonia. N Engl J Med. 2020;382:1199–1207. doi: 10.1056/NEJMoa2001316. [PMC free article] [PubMed] [CrossRef] [Google Scholar]
14. Ramaiah A, Arumugaswami V. Insights into cross-species evolution of novel human coronavirus 2019-nCoV and defining immune determinants for vaccine development. bioRxiv. 2020 Jan 30 doi: 10.1101/2020.01.29.925867. [CrossRef] [Google Scholar]
15. Chan JF, Kok KH, Zhu Z, Chu H, To KK, Yuan S. Genomic characterization of the 2019 novel human-pathogenic coronavirus isolated from a patient with atypical pneumonia after visiting Wuhan. Emerg Microbes Infect. 2020;9:221–236. doi: 10.1080/22221751.2020.1719902. [PMC free article] [PubMed] [CrossRef] [Google Scholar]
16. Wu A, Peng Y, Huang B, Ding X, Wang X, Niu P. Genome composition and divergence of the novel coronavirus (2019-nCoV) originating in China. Cell Host Microbe. 2020;27:325–328. doi: 10.1016/j.chom.2020.02.001. [PMC free article] [PubMed] [CrossRef] [Google Scholar]
17. Yuan Y, Cao D, Zhang Y, Ma J, Qi J, Wang Q. Cryo-EM structures of MERS-CoV and SARS-CoV spike glycoproteins reveal the dynamic receptor binding domains. Nat Commun. 2017;8:15092. doi: 10.1038/ncomms15092. [PMC free article] [PubMed] [CrossRef] [Google Scholar]
18. Walls AC, Xiong X, Park YJ, Tortorici MA, Snijder J, Quispe J. Unexpected receptor functional mimicry elucidates activation of coronavirus fusion. Cell. 2019;176 doi: 10.1016/j.cell.2018.12.028. 1026–39.e15. [PMC free article] [PubMed] [CrossRef] [Google Scholar]
19. Wrapp D, Wang N, Corbett KS, Goldsmith JA, Hsieh CL, Abiona O. Cryo-EM structure of the 2019-nCoV spike in the prefusion conformation. Science. 2020;367:1260–1263. doi: 10.1126/science.abb2507. [PMC free article] [PubMed] [CrossRef] [Google Scholar]
20. Li F. Structure, function, and evolution of coronavirus spike proteins. Annu Rev Virol. 2016;3:237–261. doi: 10.1146/annurev-virology-110615-042301. [PMC free article] [PubMed] [CrossRef] [Google Scholar]
21. Gui M, Song W, Zhou H, Xu J, Chen S, Xiang Y. Cryo-electron microscopy structures of the SARS-CoV spike glycoprotein reveal a prerequisite conformational state for receptor binding. Cell Res. 2017;27:119–129. doi: 10.1038/cr.2016.152. [PMC free article] [PubMed] [CrossRef] [Google Scholar]
22. Paules CI, Marston HD, Fauci AS. Coronavirus infections—more than just the common cold. JAMA. 2020 Jan 23 doi: 10.1001/jama.2020.0757. [PubMed] [CrossRef] [Google Scholar]
23. Raj VS, Mou H, Smits SL, Dekkers DH, Müller MA, Dijkman R. Dipeptidyl peptidase 4 is a functional receptor for the emerging human coronavirus-EMC. Nature. 2013;495:251–254. doi: 10.1038/nature12005. [PMC free article] [PubMed] [CrossRef] [Google Scholar]
24. Kuba K, Imai Y, Rao S, Gao H, Guo F, Guan B. A crucial role of angiotensin converting enzyme 2 (ACE2) in SARS coronavirus-induced lung injury. Nat Med. 2005;11:875–879. doi: 10.1038/nm1267. [PMC free article] [PubMed] [CrossRef] [Google Scholar]
25. Hui DS, I Azhar E, Madani TA, Ntoumi F, Kock R, Dar O. The continuing 2019-nCoV epidemic threat of novel coronaviruses to global health—the latest 2019 novel coronavirus outbreak in Wuhan, China. Int J Infect Dis. 2020;91:264–266. doi: 10.1016/j.ijid.2020.01.009. [PMC free article] [PubMed] [CrossRef] [Google Scholar]
26. Zhou P, Yang XL, Wang XG, Hu B, Zhang L, Zhang W. A pneumonia outbreak associated with a new coronavirus of probable bat origin. Nature. 2020;579:270–273. doi: 10.1038/s41586-020-2012-7. [PMC free article] [PubMed] [CrossRef] [Google Scholar]
27. Villar J, Zhang H, Slutsky AS. Lung repair and regeneration in ARDS: role of PECAM1 and Wnt signaling. Chest. 2019;155:587–594. doi: 10.1016/j.chest.2018.10.022. [PMC free article] [PubMed] [CrossRef] [Google Scholar]
28. Channappanavar R, Perlman S. Pathogenic human coronavirus infections: causes and consequences of cytokine storm and immunopathology. Semin Immunopathol. 2017;39:529–539. doi: 10.1007/s00281-017-0629-x. [PMC free article] [PubMed] [CrossRef] [Google Scholar]
29. Wang H, Ma S. The cytokine storm and factors determining the sequence and severity of organ dysfunction in multiple organ dysfunction syndrome. Am J Emerg Med. 2008;26:711–715. doi: 10.1016/j.ajem.2007.10.031. [PubMed] [CrossRef] [Google Scholar]
30. Chen N, Zhou M, Dong X, Qu J, Gong F, Han Y. Epidemiological and clinical characteristics of 99 cases of 2019 novel coronavirus pneumonia in Wuhan, China: a descriptive study. Lancet. 2020;395:507–513. doi: 10.1016/S0140-6736(20)30211-7. [PMC free article] [PubMed] [CrossRef] [Google Scholar]
31. Diao B, Wang C, Tan Y, Chen X, Liu Y, Ning L. Reduction and functional exhaustion of T cells in patients with coronavirus disease 2019 (COVID-19) medRxiv. 2020 Feb 20 doi: 10.1101/2020.02.18.20024364. [PMC free article] [PubMed] [CrossRef] [Google Scholar]
32. Chan JF, Yuan S, Kok KH, To KK, Chu H, Yang J. A familial cluster of pneumonia associated with the 2019 novel coronavirus indicating person-to-person transmission: a study of a family cluster. Lancet. 2020;395:514–523. doi: 10.1016/S0140-6736(20)30154-9. [PMC free article] [PubMed] [CrossRef] [Google Scholar]
33. Gralinski LE, Menachery VD. Return of the coronavirus: 2019-nCoV. Viruses. 2020;12 doi: 10.3390/v12020135. pii: E135. [PMC free article] [PubMed] [CrossRef] [Google Scholar]
34. Lu R, Zhao X, Li J, Niu P, Yang B, Wu H. Genomic characterisation and epidemiology of 2019 novel coronavirus: implications for virus origins and receptor binding. Lancet. 2020;395:565–574. doi: 10.1016/S0140-6736(20)30251-8. [PMC free article] [PubMed] [CrossRef] [Google Scholar]
35. Zhu N, Zhang D, Wang W, Li X, Yang B, Song J. A novel coronavirus from patients with pneumonia in China, 2019. N Engl J Med. 2020;382:727–733. doi: 10.1056/NEJMoa2001017. [PMC free article] [PubMed] [CrossRef] [Google Scholar]
36. Lam TT-Y, Shum MH-H, Zhu H-C, Tong Y-G, Ni X-B, Liao Y-S. Identifying SARS-CoV-2 related coronaviruses in Malayan pangolins. nature. 2020 doi: 10.1038/s41586-020-2169-0. [PubMed] [CrossRef] [Google Scholar]
37. Lu CW, Liu XF, Jia ZF. 2019-nCoV transmission through the ocular surface must not be ignored. Lancet. 2020;395:e39. doi: 10.1016/S0140-6736(20)30313-5. [PMC free article] [PubMed] [CrossRef] [Google Scholar]
38. Carlos WG, Dela Cruz CS, Cao B, Pasnick S, Jamil S. Novel Wuhan (2019-nCoV) coronavirus. Am J Respir Crit Care Med. 2020;201:P7–P8. doi: 10.1164/rccm.2014P7. [PubMed] [CrossRef] [Google Scholar]
39. Xia J, Tong J, Liu M, Shen Y, Guo D. Evaluation of coronavirus in tears and conjunctival secretions of patients with SARS-CoV-2 infection. J Med Virol. 2020 Feb 26 doi: 10.1002/jmv.25725. [Epub ahead of print] [PMC free article] [PubMed] [CrossRef] [Google Scholar]
40. Wang D, Hu B, Hu C, Zhu F, Liu X, Zhang J. Clinical characteristics of 138 hospitalized patients with 2019 novel coronavirus-infected pneumonia in Wuhan, China. JAMA. 2020 Feb 7 doi: 10.1001/jama.2020.1585. [Epub ahead of print] [PMC free article] [PubMed] [CrossRef] [Google Scholar]
41. Holshue ML, DeBolt C, Lindquist S, Lofy KH, Wiesman J, Bruce H. First case of 2019 novel coronavirus in the United States. N Engl J Med. 2020;382:929–936. doi: 10.1056/NEJMoa2001191. [PMC free article] [PubMed] [CrossRef] [Google Scholar]
42. Zhang H, Kang Z, Gong H, Xu D, Wang J, Li Z. The digestive system is a potential route of 2019-nCov infection: a bioinformatics analysis based on single-cell transcriptomes. bioRxiv. 2020 Jan 31 doi: 10.1101/2020.01.30.927806. [CrossRef] [Google Scholar]
43. Huang C, Wang Y, Li X, Ren L, Zhao J, Hu Y. Clinical features of patients infected with 2019 novel coronavirus in Wuhan, China. Lancet. 2020;395:497–506. doi: 10.1016/S0140-6736(20)30183-5. [PMC free article] [PubMed] [CrossRef] [Google Scholar]
44. Chen H, Guo J, Wang C, Luo F, Yu X, Zhang W. Clinical characteristics and intrauterine vertical transmission potential of COVID-19 infection in nine pregnant women: a retrospective review of medical records. Lancet. 2020;395:809–815. doi: 10.1016/S0140-6736(20)30360-3. [PMC free article] [PubMed] [CrossRef] [Google Scholar]
45. Working Group for the Prevention and Control of Neonatal 2019-nCoV Infection in the Perinatal Period of the Editorial Committee of Chinese Journal of Contemporary Pediatrics Perinatal and neonatal management plan for prevention and control of 2019 novel coronavirus infection (1st Edition) [in Chinese] Zhongguo Dang Dai Er Ke Za Zhi. 2020;22:87–90. [PMC free article] [PubMed] [Google Scholar]
46. Backer JA, Klinkenberg D, Wallinga J. Incubation period of 2019 novel coronavirus (2019-nCoV) infections among travellers from Wuhan, China, 20–28 January 2020. Euro Surveill. 2020;25 doi: 10.2807/1560-7917.ES.2020.25.5.2000062. [PMC free article] [PubMed] [CrossRef] [Google Scholar]
47. Lauer SA, Grantz KH, Bi Q, Jones FK, Zheng Q, Meredith H. The Incubation Period of Coronavirus Disease 2019 (COVID-19) From Publicly Reported Confirmed Cases: Estimation and Application. Ann Intern Med. 2020 doi: 10.7326/M20-0504. [PMC free article] [PubMed] [CrossRef] [Google Scholar]
48. Lessler J, Reich NG, Brookmeyer R, Perl TM, Nelson KE, Cummings DA. Incubation periods of acute respiratory viral infections: a systematic review. Lancet Infect Dis. 2009;9:291–300. doi: 10.1016/S1473-3099(09)70069-6. [PMC free article] [PubMed] [CrossRef] [Google Scholar]
49. Varia M, Wilson S, Sarwal S, McGeer A, Gournis E, Galanis E. Investigation of a nosocomial outbreak of severe acute respiratory syndrome (SARS) in Toronto, Canada. CMAJ. 2003;169:285–292. [PMC free article] [PubMed] [Google Scholar]
50. Assiri A, Al-Tawfiq JA, Al-Rabeeah AA, Al-Rabiah FA, Al-Hajjar S, Al-Barrak A. Epidemiological, demographic, and clinical characteristics of 47 cases of Middle East respiratory syndrome coronavirus disease from Saudi Arabia: a descriptive study. Lancet Infect Dis. 2013;13:752–761. doi: 10.1016/S1473-3099(13)70204-4. [PMC free article] [PubMed] [CrossRef] [Google Scholar]
51. Rothe C, Schunk M, Sothmann P, Bretzel G, Froeschl G, Wallrauch C. Transmission of 2019-nCoV infection from an asymptomatic contact in Germany. N Engl J Med. 2020;382:970–971. doi: 10.1056/NEJMc2001468. [PMC free article] [PubMed] [CrossRef] [Google Scholar]
52. Quilty BJ, Clifford S, Flasche S, Eggo RM, CMMID nCoV Working Group Effectiveness of airport screening at detecting travellers infected with novel coronavirus (2019-nCoV) Euro Surveill. 2020;25 doi: 10.2807/1560-7917.ES.2020.25.5.2000080. [PMC free article] [PubMed] [CrossRef] [Google Scholar]
53. Lipsitch M, Cohen T, Cooper B, Robins JM, Ma S, James L. Transmission dynamics and control of severe acute respiratory syndrome. Science. 2003;300:1966–1970. doi: 10.1126/science.1086616. [PMC free article] [PubMed] [CrossRef] [Google Scholar]
54. Liu Y, Gayle AA, Wilder-Smith A, Rocklöv J. The reproductive number of COVID-19 is higher compared to SARS coronavirus. J Travel Med. 2020;27 doi: 10.1093/jtm/taaa021. pii: taaa021. [PMC free article] [PubMed] [CrossRef] [Google Scholar]
55. Xu Z, Shi L, Wang Y, Zhang J, Huang L, Zhang C. Pathological findings of COVID-19 associated with acute respiratory distress syndrome. Lancet Respir Med. 2020 Feb 18 doi: 10.1016/S2213-2600(20)30076-X. [Epub ahead of print] [PMC free article] [PubMed] [CrossRef] [Google Scholar]
56. Ding Y, Wang H, Shen H, Li Z, Geng J, Han H. The clinical pathology of severe acute respiratory syndrome (SARS): a report from China. J Pathol. 2003;200:282–289. doi: 10.1002/path.1440. [PMC free article] [PubMed] [CrossRef] [Google Scholar]
57. Ng DL, Al Hosani F, Keating MK, Gerber SI, Jones TL, Metcalfe MG. Clinicopathologic, immunohistochemical, and ultrastructural findings of a fatal case of Middle East respiratory syndrome coronavirus infection in the United Arab Emirates, April 2014. Am J Pathol. 2016;186:652–658. doi: 10.1016/j.ajpath.2015.10.024. [PMC free article] [PubMed] [CrossRef] [Google Scholar]
58. Chung M, Bernheim A, Mei X, Zhang N, Huang M, Zeng X. CT imaging features of 2019 novel coronavirus (2019-nCoV) Radiology. 2020;295:202–207. doi: 10.1148/radiol.2020200230. [PMC free article] [PubMed] [CrossRef] [Google Scholar]
59. Shi H, Han X, Zheng C. Evolution of CT manifestations in a patient recovered from 2019 novel coronavirus (2019-nCoV) pneumonia in Wuhan, China. Radiology. 2020;295:20. doi: 10.1148/radiol.2020200269. [PMC free article] [PubMed] [CrossRef] [Google Scholar]
60. Corman VM, Landt O, Kaiser M, Molenkamp R, Meijer A, Chu DKW. Detection of 2019 novel coronavirus (2019-nCoV) by real-time RT-PCR. Euro Surveill. 2020;25 doi: 10.2807/1560-7917.ES.2020.25.3.2000045. [PMC free article] [PubMed] [CrossRef] [Google Scholar]
61. Chu DKW, Pan Y, Cheng SMS, Hui KPY, Krishnan P, Liu Y. Molecular diagnosis of a novel coronavirus (2019-nCoV) causing an outbreak of pneumonia. Clin Chem. 2020;66:549–555. [PMC free article] [PubMed] [Google Scholar]
62. To KK, Tsang OT, Chik-Yan Yip C, Chan KH, Wu TC, Chan JMC. Consistent detection of 2019 novel coronavirus in saliva. Clin Infect Dis. 2020 Feb 12 doi: 10.1093/cid/ciaa149. [Epub ahead of print] [PMC free article] [PubMed] [CrossRef] [Google Scholar]
63. Zhang F, Abudayyeh OO, Gootenberg JS. A protocol for detection of COVID-19 using CRISPR diagnostics. https://broad.io/sherlockprotocol[accessed 1 April 2020].
64. Ströher U, DiCaro A, Li Y, Strong JE, Aoki F, Plummer F. Severe acute respiratory syndrome-related coronavirus is inhibited by interferon-alpha. J Infect Dis. 2004;189:1164–1167. doi: 10.1086/382597. [PMC free article] [PubMed] [CrossRef] [Google Scholar]
65. Zorzitto J, Galligan CL, Ueng JJ, Fish EN. Characterization of the antiviral effects of interferon-α against a SARS-like coronavirus infection in vitro. Cell Res. 2006;16:220–229. doi: 10.1038/sj.cr.7310030. [PMC free article] [PubMed] [CrossRef] [Google Scholar]
66. Haagmans BL, Kuiken T, Martina BE, Fouchier RA, Rimmelzwaan GF, van Amerongen G. Pegylated interferon-α protects type 1 pneumocytes against SARS coronavirus infection in macaques. Nat Med. 2004;10:290–293. doi: 10.1038/nm1001. [PMC free article] [PubMed] [CrossRef] [Google Scholar]
67. Loutfy MR, Blatt LM, Siminovitch KA, Ward S, Wolff B, Lho H. Interferon alfacon-1 plus corticosteroids in severe acute respiratory syndrome: a preliminary study. JAMA. 2003;290:3222–3228. doi: 10.1001/jama.290.24.3222. [PubMed] [CrossRef] [Google Scholar]
68. Walmsley S, Bernstein B, King M, Arribas J, Beall G, Ruane P. Lopinavir–ritonavir versus nelfinavir for the initial treatment of HIV infection. N Engl J Med. 2002;346:2039–2046. doi: 10.1056/NEJMoa012354. [PubMed] [CrossRef] [Google Scholar]
69. Pulido F, Arribas JR, Delgado R, Cabrero E, González-García J, Pérez-Elias MJ. Lopinavir–ritonavir monotherapy versus lopinavir–ritonavir and two nucleosides for maintenance therapy of HIV. AIDS. 2008;22:F1–F9. doi: 10.1097/QAD.0b013e3282f4243b. [PubMed] [CrossRef] [Google Scholar]
70. Lin S, Shen R, Guo X. Molecular modeling evaluation of the binding abilities of ritonavir and lopinavir to Wuhan pneumonia coronavirus proteases. bioRxiv. 2020 Feb 3 doi: 10.1101/2020.01.31.929695. [CrossRef] [Google Scholar]
71. Chu CM, Cheng VC, Hung IF, Wong MM, Chan KH, Chan KS. Role of lopinavir/ritonavir in the treatment of SARS: initial virological and clinical findings. Thorax. 2004;59:252–256. doi: 10.1136/thorax.2003.012658. [PMC free article] [PubMed] [CrossRef] [Google Scholar]
72. Sheahan TP, Sims AC, Leist SR, Schäfer A, Won J, Brown AJ. Comparative therapeutic efficacy of remdesivir and combination lopinavir, ritonavir, and interferon beta against MERS-CoV. Nat Commun. 2020;11:222. doi: 10.1038/s41467-019-13940-6. [PMC free article] [PubMed] [CrossRef] [Google Scholar]
73. Zumla A, Chan JF, Azhar EI, Hui DS, Yuen K. Coronaviruses—drug discovery and therapeutic options. Nat Rev Drug Discov. 2016;15:327–347. doi: 10.1038/nrd.2015.37. [PMC free article] [PubMed] [CrossRef] [Google Scholar]
74. Wenzel RP, Edmond MB. Managing SARS amidst uncertainty. N Engl J Med. 2003;348:1947–1948. doi: 10.1056/NEJMp030072. [PubMed] [CrossRef] [Google Scholar]
75. Jones BM, Ma ES, Peiris JS, Wong PC, Ho JC, Lam B. Prolonged disturbances of in vitro cytokine production in patients with severe acute respiratory syndrome (SARS) treated with ribavirin and steroids. Clin Exp Immunol. 2004;135:467–473. doi: 10.1111/j.1365-2249.2003.02391.x. [PMC free article] [PubMed] [CrossRef] [Google Scholar]
76. Peiris JS, Lai ST, Poon LL, Guan Y, Yam LY, Lim W. Coronavirus as a possible cause of severe acute respiratory syndrome. Lancet. 2003;361:1319–1325. doi: 10.1016/s0140-6736(03)13077-2. [PMC free article] [PubMed] [CrossRef] [Google Scholar]
77. Savarino A, Di Trani L, Donatelli I, Cauda R, Cassone A. New insights into the antiviral effects of chloroquine. Lancet Infect Dis. 2006;6:67–69. doi: 10.1016/S1473-3099(06)70361-9. [PMC free article] [PubMed] [CrossRef] [Google Scholar]
78. Yan Y, Zou Z, Sun Y, Li X, Xu KF, Wei Y. Anti-malaria drug chloroquine is highly effective in treating avian influenza A H5N1 virus infection in an animal model. Cell Res. 2013;23:300–302. doi: 10.1038/cr.2012.165. [PMC free article] [PubMed] [CrossRef] [Google Scholar]
79. Wang M, Cao R, Zhang L, Yang X, Liu J, Xu M. Remdesivir and chloroquine effectively inhibit the recently emerged novel coronavirus (2019-nCoV) in vitro. Cell Res. 2020;30:269–271. doi: 10.1038/s41422-020-0282-0. [PMC free article] [PubMed] [CrossRef] [Google Scholar]
80. Khamitov RA, Loginova S, Shchukina VN, Borisevich SV, Maksimov VA, Shuster AM. Antiviral activity of arbidol and its derivatives against the pathogen of severe acute respiratory syndrome in the cell cultures [in Russian] Vopr Virusol. 2008;53:9–13. [PubMed] [Google Scholar]
81. Wang Z, Chen X, Lu Y, Chen F, Zhang W. Clinical characteristics and therapeutic procedure for four cases with 2019 novel coronavirus pneumonia receiving combined Chinese and Western medicine treatment. Biosci Trends. 2020;14:64–68. doi: 10.5582/bst.2020.01030. [PubMed] [CrossRef] [Google Scholar]
82. Zhang J, Zhou L, Yang Y, Peng W, Wang W, Chen X. Therapeutic and triage strategies for 2019 novel coronavirus disease in fever clinics. Lancet Respir Med. 2020;8:e11–e12. doi: 10.1016/S2213-2600(20)30071-0. [PMC free article] [PubMed] [CrossRef] [Google Scholar]
83. Xu X-W, Wu X-X, Jiang X-G, Xu KJ, Ying LJ, Ma CL. Clinical findings in a group of patients infected with the 2019 novel coronavirus (SARS-Cov-2) outside of Wuhan, China: retrospective case series. BMJ. 2020 doi: 10.1136/bmj.m606. 368m606. [PMC free article] [PubMed] [CrossRef] [Google Scholar]
84. de Wit E, Feldmann F, Cronin J, Jordan R, Okumura A, Thomas T. Prophylactic and therapeutic remdesivir (GS-5734) treatment in the rhesus macaque model of MERS-CoV infection. Proc Natl Acad Sci U S A. 2020;117:6771–6776. doi: 10.1073/pnas.1922083117. [PMC free article] [PubMed] [CrossRef] [Google Scholar]
85. Agostini ML, Andres EL, Sims AC, Graham RL, Sheahan TP, Lu X. Coronavirus susceptibility to the antiviral remdesivir (GS-5734) is mediated by the viral polymerase and the proofreading exoribonuclease. mBio. 2018;9 doi: 10.1128/mBio.00221-18. pii: e00221-18. [PMC free article] [PubMed] [CrossRef] [Google Scholar]
86. Hammer Q, Ruckert T, Romagnani C. Natural killer cell specificity for viral infections. Nat Immunol. 2018;19:800–808. doi: 10.1038/s41590-018-0163-6. [PubMed] [CrossRef] [Google Scholar]
87. Dai HS, Caligiuri MA. Molecular basis for the recognition of herpes simplex virus type 1 infection by human natural killer cells. Front Immunol. 2018;9:183. doi: 10.3389/fimmu.2018.00183. [PMC free article] [PubMed] [CrossRef] [Google Scholar]
88. Arase H, Mocarski ES, Campbell AE, Hill AB, Lanier LL. Direct recognition of cytomegalovirus by activating and inhibitory NK cell receptors. Science. 2002;296:1323–1326. doi: 10.1126/science.1070884. [PubMed] [CrossRef] [Google Scholar]
89. The involvement of natural killer cells in the pathogenesis of severe acute respiratory syndrome. Am J Clin Pathol. 2004;121:507–511. doi: 10.1309/WPK7-Y2XK-NF4C-BF3R. [PMC free article] [PubMed] [CrossRef] [Google Scholar]
90. Sorrento and Celularity to initiate emergency allogeneic natural killer (NK) cell therapy development for coronavirus infection. https://seekingalpha.com/pr/17762358-sorrento-and-celularity-to-initiate-emergency-allogeneic-natural-killer-nk-cell-therapy[accessed 1 April 2020].
91. Ortiz LA, Dutreil M, Fattman C, Pandey AC, Torres G, Go K. Interleukin 1 receptor antagonist mediates the antiinflammatory and antifibrotic effect of mesenchymal stem cells during lung injury. Proc Natl Acad Sci U S A. 2007;104:11002–11007. doi: 10.1073/pnas.0704421104. [PMC free article] [PubMed] [CrossRef] [Google Scholar]
92. Gupta N, Su X, Popov B, Lee JW, Serikov V, Matthay MA. Intrapulmonary delivery of bone marrow-derived mesenchymal stem cells improves survival and attenuates endotoxin-induced acute lung injury in mice. J Immunol. 2007;179:1855–1863. doi: 10.4049/jimmunol.179.3.1855. [PubMed] [CrossRef] [Google Scholar]
93. Moodley Y, Atienza D, Manuelpillai U, Samuel CS, Tchongue J, Ilancheran S. Human umbilical cord mesenchymal stem cells reduce fibrosis of bleomycin-induced lung injury. Am J Pathol. 2009;175:303–313. doi: 10.2353/ajpath.2009.080629. [PMC free article] [PubMed] [CrossRef] [Google Scholar]
94. Matthay MA, Goolaerts A, Howard JP, Lee JW. Mesenchymal stem cells for acute lung injury: preclinical evidence. Crit Care Med. 2010;38(10 Suppl):S569–S573. doi: 10.1097/CCM.0b013e3181f1ff1d. [PMC free article] [PubMed] [CrossRef] [Google Scholar]
95. Kumamoto M, Nishiwaki T, Matsuo N, Kimura H, Matsushima K. Minimally cultured bone marrow mesenchymal stem cells ameliorate fibrotic lung injury. Eur Respir J. 2009;34:740–748. doi: 10.1183/09031936.00128508. [PubMed] [CrossRef] [Google Scholar]
96. El Agha E, Kramann R, Schneider RK, Li X, Seeger W, Humphreys BD. Mesenchymal stem cells in fibrotic disease. Cell Stem Cell. 2017;21:166–177. doi: 10.1016/j.stem.2017.07.011. [PubMed] [CrossRef] [Google Scholar]
97. Zhou B, Zhong N, Guan Y. Treatment with convalescent plasma for influenza A (H5N1) infection. N Engl J Med. 2007;357:1450–1451. doi: 10.1056/NEJMc070359. [PubMed] [CrossRef] [Google Scholar]
98. van Griensven J, Edwards T, de Lamballerie X, Semple MG, Gallian P, Baize S. Evaluation of convalescent plasma for Ebola virus disease in Guinea. N Engl J Med. 2016;374:33–42. doi: 10.1056/NEJMoa1511812. [PMC free article] [PubMed] [CrossRef] [Google Scholar]
99. Rinaldo CR., Jr. Passive immunization against poliomyelitis: the Hammon gamma globulin field trials, 1951–1953. Am J Public Health. 2005;95:790–799. doi: 10.2105/AJPH.2004.040790. [PMC free article] [PubMed] [CrossRef] [Google Scholar]
100. Cheng Y, Wong R, Soo YO, Wong WS, Lee CK, Ng MH. Use of convalescent plasma therapy in SARS patients in Hong Kong. Eur J Clin Microbiol Infect Dis. 2005;24:44–46. doi: 10.1007/s10096-004-1271-9. [PMC free article] [PubMed] [CrossRef] [Google Scholar]
101. Wong SS, Yuen KY. The management of coronavirus infections with particular reference to SARS. J Antimicrob Chemother. 2008;62:437–441. doi: 10.1093/jac/dkn243. [PMC free article] [PubMed] [CrossRef] [Google Scholar]
102. Yeh KM, Chiueh TS, Siu LK, Lin JC, Chan PK, Peng MY. Experience of using convalescent plasma for severe acute respiratory syndrome among healthcare workers in a Taiwan hospital. J Antimicrob Chemother. 2005;56:919–922. doi: 10.1093/jac/dki346. [PMC free article] [PubMed] [CrossRef] [Google Scholar]
103. ter Meulen J, Bakker AB, van den Brink EN, Weverling GJ, Martina BE, Haagmans BL. Human monoclonal antibody as prophylaxis for SARS coronavirus infection in ferrets. Lancet. 2004;363:2139–2141. doi: 10.1016/S0140-6736(04)16506-9. [PMC free article] [PubMed] [CrossRef] [Google Scholar]
104. ter Meulen J, van den Brink EN, Poon LL, Marissen WE, Leung CS, Cox F. Human monoclonal antibody combination against SARS coronavirus: synergy and coverage of escape mutants. PLoS Med. 2006;3:e237. doi: 10.1371/journal.pmed.0030237. [PMC free article] [PubMed] [CrossRef] [Google Scholar]
105. Traggiai E, Becker S, Subbarao K, Kolesnikova L, Uematsu Y, Gismondo MR. An efficient method to make human monoclonal antibodies from memory B cells: potent neutralization of SARS coronavirus. Nat Med. 2004;10:871–875. doi: 10.1038/nm1080. [PMC free article] [PubMed] [CrossRef] [Google Scholar]
106. Wong SK, Li W, Moore MJ, Choe H, Farzan M. A 193-amino acid fragment of the SARS coronavirus S protein efficiently binds angiotensin-converting enzyme 2. J Biol Chem. 2004;279:3197–3201. doi: 10.1074/jbc.C300520200. [PubMed] [CrossRef] [Google Scholar]
107. Tian X, Li C, Huang A, Xia S, Lu S, Shi Z. Potent binding of 2019 novel coronavirus spike protein by a SARS coronavirus-specific human monoclonal antibody. Emerg Microbes Infect. 2020;9:382–385. doi: 10.1080/22221751.2020.1729069. [PMC free article] [PubMed] [CrossRef] [Google Scholar]
108. Cinatl J, Morgenstern B, Bauer G, Chandra P, Rabenau H, Doerr HW. Glycyrrhizin, an active component of liquorice roots, and replication of SARS-associated coronavirus. Lancet. 2003;361:2045–2046. doi: 10.1016/s0140-6736(03)13615-x. [PMC free article] [PubMed] [CrossRef] [Google Scholar]
109. Hoever G, Baltina L, Michaelis M, Kondratenko R, Baltina L, Tolstikov GA. Antiviral activity of glycyrrhizic acid derivatives against SARS-coronavirus. J Med Chem. 2005;48:1256–1259. doi: 10.1021/jm0493008. [PubMed] [CrossRef] [Google Scholar]
110. Lu H, Huo N, Wang G, Li H, Nie L, Xu X. Clinical observation of therapeutic effect of compound glycyrrhizin on SARS. China Pharmacy. 2003;10 [Google Scholar]
111. Wu C, Xu X, Lu H. Analysis of the chest X-ray manifestations in SARS patients treated with compound glycyrrhizin. China Pharmacy. 2004;1 [Google Scholar]
112. Chen H, Du Q. Potential natural compounds for preventing 2019-nCoV infection. Preprints. 2020 [Google Scholar]
113. Lin CW, Tsai FJ, Tsai CH, Lai CC, Wan L, Ho TY. Anti-SARS coronavirus 3C-like protease effects of Isatis indigotica root and plant-derived phenolic compounds. Antiviral Res. 2005;68:36–42. doi: 10.1016/j.antiviral.2005.07.002. [PMC free article] [PubMed] [CrossRef] [Google Scholar]
114. Chen F, Chan KH, Jiang Y, Kao RY, Lu HT, Fan KW. In vitro susceptibility of 10 clinical isolates of SARS coronavirus to selected antiviral compounds. J Clin Virol. 2004;31:69–75. doi: 10.1016/j.jcv.2004.03.003. [PMC free article] [PubMed] [CrossRef] [Google Scholar]
115. Chen L, Li J, Luo C, Liu H, Xu W, Chen G. Binding interaction of quercetin-3-β-galactoside and its synthetic derivatives with SARS-CoV 3CL(pro): structure–activity relationship studies reveal salient pharmacophore features. Bioorg Med Chem. 2006;14:8295–8306. doi: 10.1016/j.bmc.2006.09.014. [PMC free article] [PubMed] [CrossRef] [Google Scholar]
116. Yi L, Li Z, Yuan K, Qu X, Chen J, Wang G. Small molecules blocking the entry of severe acute respiratory syndrome coronavirus into host cells. J Virol. 2004;78:11334–11339. doi: 10.1128/JVI.78.20.11334-11339.2004. [PMC free article] [PubMed] [CrossRef] [Google Scholar]
117. Chai X, Hu L, Zhang Y, Han W, Lu Z, Ke A. Specific ACE2 expression in cholangiocytes may cause liver damage after 2019-nCoV infection. bioRxiv. 2020 Feb 4 doi: 10.1101/2020.02.03.931766. [CrossRef] [Google Scholar]118. Li Z, Wu M, Yao J, Guo J, Liao X, Song S. Caution on kidney dysfunctions of 2019-nCoV patients. medRxiv. 2020 Mar 27 doi: 10.1101/2020.02.08.20021212. [CrossRef] [Google Scholar]
119. Fan C, Li K, Ding Y, Lu WL, Wang J. ACE2 expression in kidney and testis may cause kidney and testis damage after 2019-nCoV infection. medRxiv. 2020 Feb 13 doi: 10.1101/2020.02.12.20022418. [CrossRef] [Google Scholar]
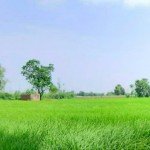